CMS e LHCb pôem o modelo standard à Prova !
Nobel Prize (5) - Gell-Mann, Leon Max Lederman, Wolfgang Pauli, Planck e Peter Higgs
Os novos resultados serão apresentados esta tarde na conferência de EPS- HEP , em Estocolmo (Suécia) é um dos mais rigorosos testes até hoje para confirmar o modelo padrão da física de partículas. CMS e LHCb experimentos do Large Hadron Collider (LHC ) vai apresentar os resultados de uma das poucas processo físico mensurável : o méson Bs decadência em dois múons .
As novas medições mostram que apenas um punhado de méson Bs decadência em um bilhão de pares de múons . Este processo é tão raro que é uma sonda extremamente sensível para revelar nova física além do Modelo Standard2 . Todos os desvios padrão das previsões do modelo seria um sinal claro de algo novo.
Os resultados apresentados por ambas as experiências têm um significado estatístico muito elevado (mais do que quatro sigma para cada experimento ) . Eles concordam bem com o Modelo Padrão.
![]() |
Colisão de protões no detetor CMS produziram uma particula B que se desintegra em dois muões (linhas vermelhas) |
"Este é um grande resultado para LHCb , disse o porta-voz da colaboração, Pierluigi Campana.C é precisamente para este tipo de ação que foi construído LHCb . Este resultado mostra que o teste que nos submetemos ao Modelo Padrão é realmente o mais rigoroso já feito para energias do LHC , e no momento, ele consegue com folga . "
O Modelo Padrão tem sido desenvolvido ao longo de mais de 40 anos. Há uma teoria que prevê uma forma extraordinariamente eficaz para multar o comportamento das partículas fundamentais , e foi testado experimentalmente com grande precisão. Mas o Modelo Padrão ainda não revelou tudo : não explicar a gravidade , por exemplo, e não descrever o universo chamado de "dark ".
Apenas cerca de 5 % do nosso Universo é o tipo de matéria visível descrito pelo Modelo Padrão. O resto é feito de matéria escura e energia escura , cuja presença é inferida a partir da influência que eles têm sobre o , a matéria visível comum.
"Este é um processo que os físicos de partículas estão tentando ser por 25 anos , disse o porta-voz do CMS , Joe Incandela . Isso demonstra as capacidades surpreendentes dos experimentos do LHC , como CMS , que foram capazes de detectar um processo muito raro , envolvendo uma partícula cuja massa é cerca de 1000 vezes menor que as massas das partículas mais pesadas que estamos procurando. "
Se estes novos resultados confirmam ainda mais o Modelo Padrão , a nova física ainda não disse sua última palavra. Uma das teorias mais populares é a supersimetria , SUSY abreviado . Postula-se a existência de um novo tipo de partícula para cada partícula do modelo padrão que se sabe, e algumas destas partículas possuem propriedades adequadas para a composição de a maior parte do universo escuro.
Existem muitos modelos SUSY no estudo , e SUSY é apenas uma das muitas possibilidades teóricas para a descoberta da física além do Modelo Padrão . Graças às medidas apresentadas hoje , os físicos são capazes de distinguir entre esses modelos. Muitos são incompatíveis com as novas medidas e pode, portanto, ser desconsiderada. Teóricos , assim, ser capaz de se concentrar no outro.
Se estes novos resultados confirmam ainda mais o Modelo Padrão , a nova física ainda não disse sua última palavra. Uma das teorias mais populares é a supersimetria , SUSY abreviado . Postula-se a existência de um novo tipo de partícula para cada partícula do modelo padrão que se sabe, e algumas destas partículas possuem propriedades adequadas para a composição de a maior parte do universo escuro.
Existem muitos modelos SUSY no estudo , e SUSY é apenas uma das muitas possibilidades teóricas para a descoberta da física além do Modelo Padrão . Graças às medidas apresentadas hoje , os físicos são capazes de distinguir entre esses modelos. Muitos são incompatíveis com as novas medidas e pode, portanto, ser desconsiderada. Teóricos , assim, ser capaz de se concentrar no outro.
The six quarks. Why "Quark"?
The name "quark" was taken by Murray Gell-Mann from the book "Finnegan's Wake" by James Joyce. The line "Three quarks for Muster Mark..." appears in the fanciful book. Gell-Mann received the 1969 Nobel Prize for his work in classifying elementary particles.
Up and Down Quarks
The up and down quarks are the most common and least massive quarks, being the constituents of protons and neutrons and thus of most ordinary matter.
![]() |
The fact that the free neutron decays
![]()
and nuclei decay by beta decay in processes like
![]()
is thought to be the result of a more fundamental quark process
![]() |
The Strange Quark
In 1947 during a study of cosmic ray interactions, a product of a proton collision with a nucleus was found to live for much longer time than expected: 10-10 seconds instead of the expected 10-23 seconds! This particle was named the lambda particle (Λ0) and the property which caused it to live so long was dubbed "strangeness" and that name stuck to be the name of one of the quarks from which the lambda particle is constructed. The lambda is a baryon which is made up of three quarks: an up, a down and a strange quark.
The shorter lifetime of 10-23 seconds was expected because the lambda as a baryon participates in the strong interaction, and that usually leads to such very short lifetimes. The long observed lifetime helped develop a new conservation law for such decays called the "conservation of strangeness". The presence of a strange quark in a particle is denoted by a quantum number S=-1. Particle decay by the strong or electromagnetic interactions preserve the strangeness quantum number.
The decay process for the lambda particle must violate that rule, since there is no lighter particle which contains a strange quark - so the strange quark must be transformed to another quark in the process. That can only occur by the weak interaction, and that leads to a much longer lifetime. The decay processes show that strangeness is not conserved:
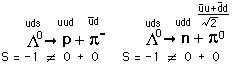
The quark transformations necessary to accomplish these decay processes can be visualized with the help of Feynmann diagrams.
![]() |
The omega-minus, a baryon composed of three strange quarks, is a classic example of the need for the property called "color" in describing particles.
Since quarks are fermions with spin 1/2, they must obey the Pauli exclusion principle and cannot exist in identical states. So with three strange quarks, the property which distinguishes them must be capable of at least three distinct values.
|
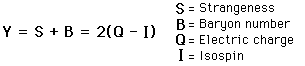
Isospin and either hypercharge or strangeness are the quantum numbers often used to draw particle diagrams for the hadrons.
The Charm Quark
In 1974 a meson called the J/Psi particle was discovered. With a mass of 3100 MeV, over three times that of the proton, this particle was the first example of another quark, called the charm quark. The J/Psi is made up of a charm-anticharm quark pair.
The lightest meson which contains a charm quark is the D meson. It provides interesting examples of decay since the charm quark must be transformed into a strange quark by the weak interaction in order for it to decay.
The lightest meson which contains a charm quark is the D meson. It provides interesting examples of decay since the charm quark must be transformed into a strange quark by the weak interaction in order for it to decay.
One baryon with a charm quark is a called a lambda with symbol Λ+c . It has a composition udc and a mass of 2281 MeV/c2.
The Top Quark
Convincing evidence for the observation of the top quark was reported by Fermilab 's Tevatron facility in April 1995. The evidence was found in the collision products of 0.9 TeV protons with equally energetic antiprotons in the proton-antiproton collider. The evidence involved analysis of trillions of 1.8 TeV proton-antiproton collisions. The Collider Detector Facility group had found 56 top candidates over a predicted background of 23 and the D0 group found 17 events over a predicted background of 3.8.
The value for the top quark mass from the combined data of the two groups after the completion of the run was 174.3 +/- 5.1 GeV. This is over 180 times the mass of a proton and about twice the mass of the next heaviest fundamental particle, the Z0 vector boson at about 93 GeV. The interaction is envisioned as follows:
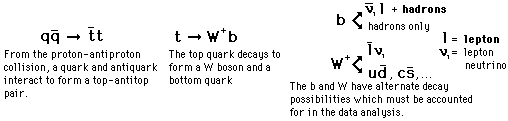
Confinement of Quarks
How can one be so confident of the quark model when no one has ever seen an isolated quark? There are good reasons for the lack of direct observation. Apparently the color force does not drop off with distance like the other observed forces. It is postutated that it may actually increase with distance at the rate of about 1 GeV per fermi.
A free quark is not observed because by the time the separation is on an observable scale, the energy is far above the pair production energy for quark-antiquark pairs. For the U and D quarks the masses are 10s of MeV so pair production would occur for distances much less than a fermi. You would expect a lot of mesons (quark-antiquark pairs) in very high energy collision experiments and that is what is observed.
Basically, you can't see an isolated quark because the color force does not let them go, and the energy required to separate them produces quark-antiquark pairs long before they are far enough apart to observe separately.
One kind of visualization of quark confinement is called the "bag model". One visualizes the quarks as contained in an elastic bag which allows the quarks to move freely around, as long as you don't try to pull them further apart. But if you try to pull a quark out, the bag stretches and resists.
Another way of looking at quark confinement is expressed by Rohlf. "When we try to pull a quark out of a proton, for example by striking the quark with another energetic particle, the quark experiences a potential energy barrier from the strong interaction that increases with distance." As the example of alpha decay demonstrates, having a barrier higher than the particle energy does not prevent the escape of the particle - quantum mechanical tunneling gives a finite probability for a 6 MeV alpha particle to get through a 30 MeV high energy barrier. But the energy barrier for the alpha particle is thin enough for tunneling to be effective. In the case of the barrier facing the quark, the energy barrier does not drop off with distance, but in fact increases.
The Bottom Quark
In 1977, an experimental group at Fermilab led by Leon Lederman discovered a new resonance at 9.4 GeV/c^2 which was interpreted as a bottom-antibottom quark pair and called the Upsilon meson. From this experiment, the mass of the bottom quark is implied to be about 5 GeV/c^2. The reaction being studied was

where N was a copper or platinum nucleus. The spectrometer had a muon-pair mass resolution of about 2%, which allowed them to measure an excess of events at 9.4 GeV/c^2. This resonance has been subsequently studied at other accelerators with a detailed investigation of the bound states of the bottom-antibottom meson.
Pentaquark
In 2003 came experimental evidence of a five-quark combination which is being called a pentaquark. Strong evidence for the pentaquark came from experiments at the Jefferson Lab in Newport News, Virginia during 2003. The experiments involved multi-GeV photons impacting a deuterium target. The evidence showed a five-quark baryon state at a mass of 1.54 GeV with a narrow width of 22 MeV.
The new particle is being called Theta-plus, with a composition of two up quarks, two down quarks and an anti-strange quark. The combination of quark charges +2/3(u), -1/3 (d) and 1/3 (anti-strange) gives a net charge of +1. The lifetime of the particle is about 10-20 seconds. The decay is classified as a strong interaction decay to a neutron and a K+ meson
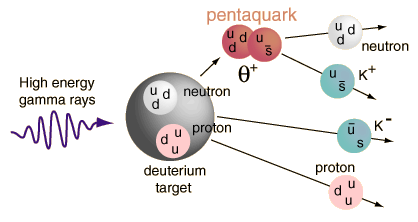
The Jefferson Lab results corroborated the growing body of evidence for the pentaquark from the last few years. Work done by a Japanese team led by Takashi Nakano of Osaka University involved bombarding a carbon target with X-rays and led to a report of pentaquark evidence in 2002. A retrospective study of 1986 bubble chamber experiments at ITEP in Moscow also revealed a 1.54 GeV peak. A review of data taken in 1997/98 at the ELSA accelerator in Bonn, Germany showed evidence of a particle of mass 1.54 GeV and width less than 25 MeV. The existence of the pentaquark was hypothesized by Maxim Polyakov, Dmitri Diakonov, and Victor Petrov at the Petersburg Nuclear Physics Institute in Russia in 1997.
While the postulated pentaquark has made an interesting story, continued experimentation has not borne it out. The 2006 Particle Data Group publication expressed its pessimism with the statement :
"There has not been a high-statistics confirmation of any of the original experiments that claimed to see the θ+; there have been two high-statistics repeats from Jefferson Lab that have clearly shown the original positive claims in those two cases to be wrong; there have been a number of other high-statistics experiments, none of which have found any evidence for the θ+; and all attempts to confirm the two other claimed pentaquark states have led to negative results. The conclusion that pentaquarks in general, and the θ+, in particular, do not exist, appears compelling."
The statement in the 2008 Summary was even stronger, and the issue appears to have been dropped from the 2010 Summary, so the consensus seems strong that this particular quark combination has not been observed.
Leptons
Leptons and quarks are the basic building blocks of matter, i.e., they are seen as the "elementary particles". There are six leptons in the present structure, the electron, muon, and tau particles and their associated neutrinos. The different varieties of the elementary particles are commonly called "flavors", and the neutrinos here are considered to have distinctly different flavor.
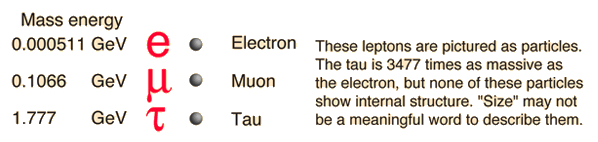
Important principles for all particle interactions are the conservation of lepton number and the the conservation of baryon number.
Now that we have experimental evidence for six leptons, a relevant question is "Are there more?". The present standard model assumes that there are no more than three generations. One of the pieces of experimental evidence for that is the measured hydrogen/helium abundance ratio in the universe. When the process of nucleosynthesis from the big bang is modeled, the number of types of neutrinos affects the abundance of helium. The observed abundance agrees with three types of neutrinos.
Electron and Positron
As one of the leptons, the electron is viewed as one of the fundamental particles. It is a fermion of spin 1/2 and therefore constrained by the Pauli exclusion principle, a fact that has key implications for the building up of the periodic table of elements.
The electron's antiparticle, the positron, is identical in mass but has a positive charge. If an electron and a positron encounter each other,they will annihilate with the production of two gamma-rays. On the other hand, one of the mechanisms for the interaction of radiation with matter is the pair production of an electron-positron pair. Associated with the electron is a the electron neutrino
.
Particle
|
Symbol
|
Anti-
particle
| MeV/c2 |
L(e)
|
L(muon)
|
L(tau)
| (seconds) |
(Electron) |
Muon
The muon is a lepton which decays to form an electron or positron.
The fact that the above decay is a three-particle decay is an example of the conservation of lepton number; there must be one electron neutrino and one muon neutrino or antineutrino in the decay.
The lifetime of the muon is 2.20 microseconds. The muon is produced in the upper atmosphere by the decay of pions produced by cosmic rays:

Measuring the flux of muons of cosmic ray origin at different heights above the earth is an important time dilation experiment in relativity.
Muons make up more than half of the cosmic radiation at sea level, the remainder being mostly electrons, positrons and photons from cascade events.(Richtmyer) The average sea level muon flux is about 1 muon per square centimeter per minute.
Particle
|
Symbol
|
Anti-
particle
| MeV/c2 |
L(e)
|
L(muon)
|
L(tau)
| (seconds) |
(Muon) |
Tau
The tau is the most massive of the leptons, having a rest mass some 3490 times the mass of the electron, also a lepton. Its mass is some 17 times that of the muon, the other massive lepton.
Particle
|
Symbol
|
Anti-
particle
| MeV/c2 |
L(e)
|
L(muon)
|
L(tau)
| (seconds) |
(Tau) |
Electron, Muon, and Tau Neutrinos
The massive leptons are the electron, muon and tau, and each of them has an associated neutrino. Most experiments involving neutrinos involve electron neutrinos which are much more common in our low-energy world, but some current neutrino detectors are sensitive to the other two as well. The current generation of neutrino detectors such as the Super-Kamiokande can detect and distinguish between electron and muon neutrinos.
When a muon neutrino interacts with a nucleus, it can produce an energetic muon which travels only a short distance, emitting a sharply outlined cone of Cerenkov radiation which can be detected by photomultiplier tubes. An electron neutrino interaction can produce an energetic electron, but the Cerenkov cone from this interaction differs significantly from that of the muon. The electron generates a shower of electrons and positrons, each with its own Cerenkov cone.
This smears out the circle of light which hits the detectors; the diffuse circle at the photomultipliers is the signature of the electron neutrino. Tau neutrinos are not detected by these detectors because the neutrino energies are not sufficient to produce tau particles (which are about 3500 times the mass of an electron).
The first clear experimental evidence for the difference between electron and muon neutrinos came from an experiment conducted at Brookhaven in 1962. The two reactions indicated would have been equally probable if electron and muon neutrinos were the same.
The first clear experimental evidence for the difference between electron and muon neutrinos came from an experiment conducted at Brookhaven in 1962. The two reactions indicated would have been equally probable if electron and muon neutrinos were the same.
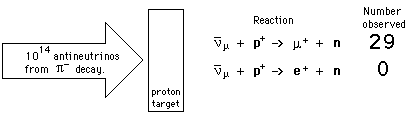
Since the electron and muon neutrinos are distinct, the second reaction above
The history of a particle that appeared to have no charge and no mass is an interesting one. The electron neutrino (a lepton) was first postulated in 1930 by Wolfgang Pauli to explain why the electrons in beta decay were not emitted with the full reaction energy of the nuclear transition. The apparent violation of conservation of energy and momentum was most easily avoided by postulating another particle. Enrico Fermi called the particle a neutrino and developed a theory of beta decay based on it, but it was not experimentally observed until 1956.
This elusive particle, with no charge and almost no mass, could penetrate vast thicknesses of material without interaction. The mean free path of a neutrino in water would be on the order of 10x the distance from the Earth to the Sun. In the standard Big Bang model, the neutrinos left over from the creation of the universe are the most abundant particles in the universe. This remnant neutrino density is put at 100 per cubic centimeter at an effective temperature of 2K (Simpson).
The background temperature for neutrinos is lower than that for the microwave background(2.7K) because the neutrino transparency point came earlier. The sun emits vast numbers of neutrinos which can pass through the earth with little or no interaction. This leads to the statement "Solar neutrinos shine down on us during the day, and shine up on us during the night!" . Bahcall's modeling of the solar neutrino flux led to the prediction of about 5 x 106 neutrinos/cm2s.
A remarkable opportunity for observing neutrinos came with Supernova 1987A when the Japanese observing team detected neutrinos almost coincident with the discovery of the light from the supernova.
Neutrinos interact only by the weak interaction. Their interactions are usually represented in terms of Feynman diagrams
A remarkable opportunity for observing neutrinos came with Supernova 1987A when the Japanese observing team detected neutrinos almost coincident with the discovery of the light from the supernova.
Neutrinos interact only by the weak interaction. Their interactions are usually represented in terms of Feynman diagrams
No definite mass has been measured for the neutrino, and the standard comment about most experiments is "the results are consistent with zero mass for the neutrino". But this raises certain theoretical problems and there have been many attempts to set a range for the mass of the neutrino. Since its mass is evidently very small, if non-zero, the mass is usually stated in terms of its energy equivalent in electron volts. Most experiments conclude that the mass equivalent of the neutrino is less than 50 eV.
One of the recent pieces of information about neutrino mass came from the neutrinos observed from Supernova 1987A. Ten neutrinos arrived within 15 seconds of each other after traveling 180,000 light years, and they differed by a up to factor of three in energy. This limits the neutrino rest mass energy to less than about 30 eV (Rohlf).
New experimental evidence from the Super-Kamiokande neutrino detector in Japan represents the strongest evidence to date that the mass of the neutrino is non-zero. Models of atmospheric cosmic ray interactions suggest twice as many muon neutrinos as electron neutrinos, but the measured ratio was only 1.3:1. The interpretation of the data suggested a mass difference between electron and muon neutrinos of 0.03 to 0.1 eV. Presuming that the muon neutrino would be much more massive than the electron neutrino, then this implies a muon neutrino mass upper bound of about 0.1 eV.
The recent neutrino measurements at the Sudbury Neutrino Observatory are consistent with the modeled total neutrino flux and add evidence for neutrino oscillation, a process which can only occur if the neutrinos have mass..
Gluons
Gluons are the exchange particles for the color force between quarks, analogous to the exchange of photons in the electromagnetic force between two charged particles. The gluon can be considered to be the fundamental exchange particle underlying the strong interaction between protons and neutrons in a nucleus. That short-range nucleon-nucleon interaction can be considered to be a residual color force extending outside the boundary of the proton or neutron. That strong interaction was modeled by Yukawa as involving an exchange of pions, and indeed the pion range calculation was helpful in developing our understanding of the strong force.
Gluon interactions are often represented by a Feynman diagram. Note that the gluon generates a color change for the quarks. The gluons are in fact considered to be bi-colored, carrying a unit of color and a unit of anti-color as suggested in the diagram at right. The gluon exchange picture there converts a blue quark to a green one and vice versa.
The range of the strong force is limited by the fact that the gluons interact with each other as well as with quarks in the context of quark confinement. These properties contrast them with photons, which are massless and of infinite range. The photon does not carry electric charge with it, while the gluons do carry the "color charge".
| ![]() |
Within their range of about a fermi, the gluons can interact with each other, and can produce virtual quark-antiquark pairs. The property of interaction with each other is very different from the other exchange particles, and raises the possibility of gluon collections referred to as "glueballs". The internal state of a hadron is viewed as composed of a fixed net number of quarks, but with a dynamic cloud of gluons and quark-antiquark pairs in equilibrium.
Feynman Diagrams
Feynman diagrams are graphical ways to represent exchange forces. Each point at which lines come together is called a vertex, and at each vertex one may examine the conservation laws which govern particle interactions. Each vertex must conserve charge, baryon number and lepton number.
Developed by Feynman to decribe the interactions in quantum electrodynamics (QED), the diagrams have found use in describing a variety of particle interactions. They are spacetime diagrams, ct vs x. The time axis points upward and the space axis to the right. (Particle physicists often reverse that orientation.)
Particles are represented by lines with arrows to denote the direction of their travel, with antiparticles having their arrows reversed. Virtual particles are represented by wavy or broken lines and have no arrows. All electromagnetic interactions can be described with combinations of primitive diagrams like this one.
| ![]() |
![]() |
Only lines entering or leaving the diagram represent observable particles. Here two electrons enter, exchange a photon, and then exit. The time and space axes are usually not indicated. The vertical direction indicates the progress of time upward, but the horizontal spacing does not give the distance between the particles.
|
Other electromagnetic process can be represented, as in the examples below. A backward arrow represents the antiparticle, in these cases a positron. Keep in mind that time progresses upward, and that a downward arrow is not a particle progressing downward, but an antiparticle progressing upward ( forward in time).
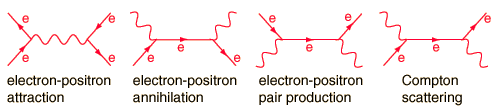
After being introduced for electromagnetic processes, Feynman diagrams were developed for the weak and strong interactions as well. Forms of primitive vertices for these three interactions are
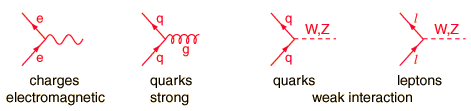
Particle interactions can be represented by diagrams with at least two vertices. They can be drawn for protons, neutrons, etc. even though they are composite objects and the interaction can be visualized as being between their constituent quarks.
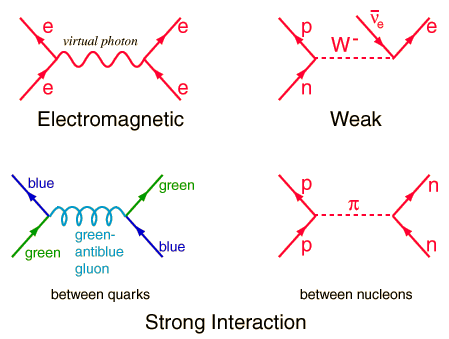
The W and Z particles are the massive exchange particles which are involved in the nuclear weak interaction, the weak force between electrons and neutrinos. They were predicted by Weinberg, Salam, and Glashow in 1979 and measured at CERN in 1982. The prediction included a prediction of the masses of these particles as a part of the unified theory of the electromagnetic and weak forces, the electroweak unification. "If the weak and electromagnetic forces are essentially the same, then they must also have the same strength.
The fact that the experimentally observed strengths seem quite different is attributed to the masses of the W and Z particles-under certain conditions a force of large strength can have the appearance of a force of small strength if the particle that carries the force is very massive. Theoretical calculations show that at a fundamental level the weak and electromagnetic forces have the same strength if the W and Z particles have masses of 80 and 90 GeV respectively." The masses measured at CERN were 82 and 93 GeV, a brilliant confirmation of the electroweak unification.
The experiments at CERN detected a total of 10 W bosons and 4 Z bosons. In the extended experiment at Fermilab's Tevatron known as "Run 1" (1992-96), the D0 detector facility measured over 100,000 W particles. The D0 value for the mass of the W is 80.482 +/- 0.091 GeV. Current values combining the experiments at the Tevatron and at CERN's LEP electron-positron collider are MW = 80.41 +/- 0.18 GeV and MZ = 91.1884 +/- 0.0022 GeV.
Photons: The Quanta of Light
According to the Planck hypothesis, all electromagnetic radiation is quantized and occurs in finite "bundles" of energy which we call photons. The quantum of energy for a photon is not Planck's constant h itself, but the product of h and the frequency. The quantization implies that a photon of blue light of given frequency or wavelength will always have the same size quantum of energy. For example, a photon of blue light of wavelength 450 nm will always have 2.76 eV of energy. It occurs in quantized chunks of 2.76 eV, and you can't have half a photon of blue light - it always occurs in precisely the same sized energy chunks.
But the frequency available is continuous and has no upper or lower bound, so there is no finite lower limit or upper limit on the possible energy of a photon. On the upper side, there are practical limits because you have limited mechanisms for creating really high energy photons. Low energy photons abound, but when you get below radio frequencies, the photon energies are so tiny compared to room temperature thermal energy that you really never see them as distinct quantized entities - they are swamped in the background. Another way to say it is that in the low frequency limits, things just blend in with the classical treatment of things and a quantum treatment is not necessary.
The experiments at CERN detected a total of 10 W bosons and 4 Z bosons. In the extended experiment at Fermilab's Tevatron known as "Run 1" (1992-96), the D0 detector facility measured over 100,000 W particles. The D0 value for the mass of the W is 80.482 +/- 0.091 GeV. Current values combining the experiments at the Tevatron and at CERN's LEP electron-positron collider are MW = 80.41 +/- 0.18 GeV and MZ = 91.1884 +/- 0.0022 GeV.
Photons: The Quanta of Light
According to the Planck hypothesis, all electromagnetic radiation is quantized and occurs in finite "bundles" of energy which we call photons. The quantum of energy for a photon is not Planck's constant h itself, but the product of h and the frequency. The quantization implies that a photon of blue light of given frequency or wavelength will always have the same size quantum of energy. For example, a photon of blue light of wavelength 450 nm will always have 2.76 eV of energy. It occurs in quantized chunks of 2.76 eV, and you can't have half a photon of blue light - it always occurs in precisely the same sized energy chunks.
But the frequency available is continuous and has no upper or lower bound, so there is no finite lower limit or upper limit on the possible energy of a photon. On the upper side, there are practical limits because you have limited mechanisms for creating really high energy photons. Low energy photons abound, but when you get below radio frequencies, the photon energies are so tiny compared to room temperature thermal energy that you really never see them as distinct quantized entities - they are swamped in the background. Another way to say it is that in the low frequency limits, things just blend in with the classical treatment of things and a quantum treatment is not necessary.
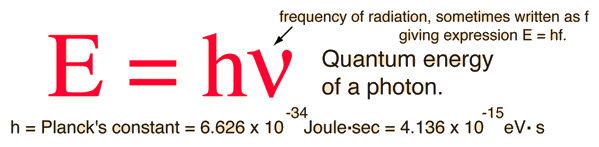
The Planck Hypothesis
In order to explain the frequency distribution of radiation from a hot cavity (blackbody radiation) Planck proposed the ad hoc assumption that the radiant energy could exist only in discrete quanta which were proportional to the frequency. This would imply that higher modes would be less populated and avoid the ultraviolet catastrophe of the Rayleigh-Jeans Law.
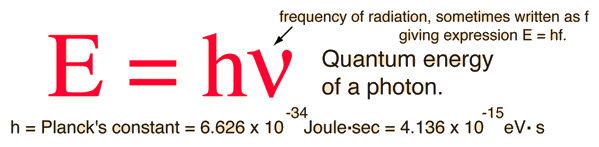
The quantum idea was soon seized to explain the photoelectric effect, became part of the Bohr theory of discrete atomic spectra, and quickly became part of the foundation of modern quantum theory.
The Higgs Boson
All the known forces in the universe are manifestations of four fundamental forces, the strong, electromagnetic, weak, and gravitational forces. But why four? Why not just one master force? Those who joined the quest for a single unified master force declared that the first step toward unification had been achieved with the discovery of the discovery of the W and Z particles, the intermediate vector bosons, in 1983.
All the known forces in the universe are manifestations of four fundamental forces, the strong, electromagnetic, weak, and gravitational forces. But why four? Why not just one master force? Those who joined the quest for a single unified master force declared that the first step toward unification had been achieved with the discovery of the discovery of the W and Z particles, the intermediate vector bosons, in 1983.
This brought experimental verification of particles whose prediction had already contributed to the Nobel prize awarded to Pauli in 1979. Combining the weak and electromagnetic forces into a unified "electroweak" force, these great advances in both theory and experiment provide encouragement for moving on to the next step, the "grand unification" necessary to include the strong interaction.
While electroweak unification was hailed as a great step forward, there remained a major conceptual problem. If the weak and electromagnetic forces are part of the same electroweak force, why is it that the exchange particle for the electromagnetic interaction, the photon, is massless while the W and Z have masses more than 80 times that of a proton!
While electroweak unification was hailed as a great step forward, there remained a major conceptual problem. If the weak and electromagnetic forces are part of the same electroweak force, why is it that the exchange particle for the electromagnetic interaction, the photon, is massless while the W and Z have masses more than 80 times that of a proton!
The electromagnetic and weak forces certainly do not look the same in the present low temperature universe, so there must have been some kind of spontaneous symmetry breaking as the hot universe cooled enough that particle energies dropped below 100 GeV. The theories attribute the symmetry-breaking to a field called the Higgs field, and it requires a new boson, the Higgs boson, to mediate it.
![]() Illustration courtesy Fermilab, D0 Experiment. |
Early formulation of the theories estimated that the Higgs boson would have mass energy in excess of 1 TeV, making the energies for discovery almost unattainable on the earth. Now, since the discovery of the top quark, there is tantalizing evidence that the Higgs boson may have energies in the range of a few hundred GeV and therefore within the range of present day accelerators.
At Fermilab, data from the D0 detector facility is used with the masses of the W and the T quark to estimate the mass of the Higgs boson. Suggestions that it may have a mass below 200 GeV have made it one of the high priorities for high energy physics.
|
Mesons
Mesons are intermediate mass particles which are made up of a quark-antiquark pair. Three quark combinations are called baryons. Mesons are bosons, while the baryons are fermions. Recent experimental evidence shows the existence of five-quark combinations which are being called pentaquarks.

Particle
|
Symbol
|
Anti-
particle
|
Makeup
| MeV/c2 |
S
|
C
|
B
|
Lifetime
| |
0
| x10-8 | ||||||||
![]() |
0
| x10-16 | |||||||
0
| x10-8 | ||||||||
0
| x10-10 | ||||||||
0
| x10-8 | ||||||||
0
| |||||||||
0
| |||||||||
0
| x10-23 | ||||||||
0
| x10-23 | ||||||||
0
| x10-22 | ||||||||
0
| x10-23 | ||||||||
0
| x10-13 | ||||||||
0
| x10-13 | ||||||||
0
| x10-13 | ||||||||
0
| x10-20 | ||||||||
-1
| x10-12 | ||||||||
-1
| x10-12 | ||||||||
-1
| |||||||||
0
| x10-20 |
Mesons are intermediate mass particles which are made up of a quark-antiquark pair. Three quark combinations are called baryons. Mesons are bosons, while the baryons are fermions.
1* The neutral Kaons K0s and K0L represent symmetric and antisymmetric mixtures of the quark combinations down-antistrange and antidown-strange.
2* The neutral eta meson is considered to be a quark combination
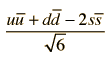
Note: In the table, antiquarks are indicated by an underline, e.g., ud means a combination of an up and an antidown quark.
Most of the listed decay times are in the range 10-13 to 10-8 s which indicates decay by the weak interaction. These decays involve quark transformations which can be represented by Feynman diagrams.
pion
Particle
|
Symbol
|
Anti-
particle
|
Makeup
| MeV/c2 |
S
|
C
|
B
|
Lifetime
| |
0
| x10-8 | ||||||||
![]() |
0
| x10-16 |
The neutral pion decays to an electron, positron, and gamma ray by the electromagnetic interaction on a time scale of about 10-16 seconds. The positive and negative pions have longer lifetimes of about 2.6 x 10-8 s.
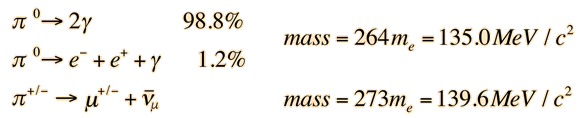
The negative pion decays into a muon and a muon antineutrino as illustrated below. This decay is puzzling upon first examination because the decay into an electron plus an electron antineutrino yields much more energy. Usually the pathway with the greatest energy yield is the preferred pathway. This suggests that some symmetry is acting to inhibit the electron decay pathway.
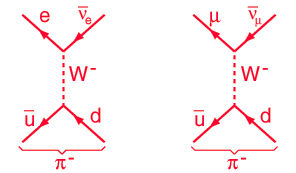
The symmetry which suppresses the electron pathway is that of angular momentum, as described by Griffiths. Since the negative pion has spin zero, the electron and antineutrino must be emitted with opposite spins to preserve net zero spin. But the antineutrino is always right-handed, so this implies that the electron must be emitted with spin in the direction of its linear momentum (i.e., also right-handed).
But if the electron were massless, it would (like the neutrino) only exist as a left-handed particle, and the electron pathway would be completely prohibited. So the suppression of the electron pathway is attributed to the fact that the electron's small mass greatly favors the left-handed symmetry, thus inhibiting the decay. Weak interaction theory predicts that the fraction of muons decaying into electrons should be 1.28 x 10-4 and the measured branching ratio is 1.23 +/- 0.02 x 10-4.
The pion, being the lightest meson, can be used to predict the maximum range of the strong interaction. The strong interaction properties of the three pions are identical. The connection between pions and the strong force was proposed by Hideki Yukawa. Yukawa worked out a potential for the force and predicted its mass based on the uncertainty principle from measurements of the apparent range of the strong force in nuclei.
Being composed of an up and an antidown quark, the positive pion would be expected to have a mass about 2/3 that of a proton, yet it's mass is only about 1/6 of that of the proton! This is an example of how hadron masses depend upon the dynamics inside the particle, and not just upon the quarks contained.
The pion is a meson. The π+ is considered to be made up of an up and an anti-down quark. The neutral pion is considered to be a combination
![]() | ![]() |
Pions interact with nuclei and transform a neutron to a proton or vice versa:

The pions π+ and π- have spin zero and negative intrinsic parity (Rohlf Sec 17-2).
The psi/J Particle
Particle
|
Symbol
|
Anti-
particle
|
Makeup
| MeV/c2 |
S
|
C
|
B
|
Lifetime
| |
0
| x10-20 |
The psi/J particle is a meson which was discovered in 1974 by experimenters at Stanford (Richter) and Brookhaven National Laboratory (Ting). Slightly more than three times as massive as the proton, this particle decayed slowly and didn't fit into the framework of the up, down, and strange quarks. It is considered to be a charm-anticharm quark pair and was the first firm experimental evidence for the fourth quark. Richter and Ting shared the 1976 Nobel Prize for their discovery.
The Upsilon Particle
Particle
|
Symbol
|
Anti-
particle
|
Makeup
| MeV/c2 |
S
|
C
|
B
|
Lifetime
| |
0
| x10-20 |
The upsilon particle is a meson which was discovered at Fermilab in 1977. It appeared as another long-lived particle which didn't fit into the framework of the first four quarks, the up,down, strange, and charm quarks. It is taken as a botton-antibottom quark pair and was the first experimental evidence of the fifth quark.
Hadrons
Particles that interact by the strong interaction are called hadrons. This general classification includes mesons and baryons but specifically excludes leptons, which do not interact by the strong force. The weak interaction acts on both hadrons and leptons.
Hadrons are viewed as being composed of quarks, either as quark-antiquark pairs (mesons) or as three quarks (baryons). There is much more to the picture than this, however, because the constituent quarks are surrounded by a cloud of gluons, the exchange particles for the color force.
Recent experimental evidence shows the existence of five-quark combinations which are being called pentaquarks.
Hadrons are viewed as being composed of quarks, either as quark-antiquark pairs (mesons) or as three quarks (baryons). There is much more to the picture than this, however, because the constituent quarks are surrounded by a cloud of gluons, the exchange particles for the color force.
Recent experimental evidence shows the existence of five-quark combinations which are being called pentaquarks.
Baryons
Baryons are massive particles which are made up of three quarks in the standard model. This class of particles includes the proton and neutron. Other baryons are the lambda, sigma, xi, and omega particles. Baryons are distinct from mesons in that mesons are composed of only two quarks. Baryons and mesons are included in the overall class known as hadrons, the particles which interact by the strong force. Baryons are fermions, while the mesons are bosons. Besides charge and spin (1/2 for the baryons), two other quantum numbers are assigned to these particles: baryon number (B=1) and strangeness (S), which in the chart can be seen to be equal to -1 times the number of strange quarks included.
The conservation of baryon number is an important rule for interactions and decays of baryons. No known interactions violate conservation of baryon number. Recent experimental evidence shows the existence of five-quark combinations which are being called pentaquarks. The pentaquark would be included in the classification of baryons, albeit an "exotic" one. The pentaquark is composed of four quarks and an antiquark, like a combination of an ordinary baryon plus a meson.
Table of Baryons
Particle
|
Symbol
|
Makeup
|
Rest mass
MeV/c2
|
Spin
|
B
|
S
| (seconds> | |
x10-10 | ||||||||
x10-10 | ||||||||
x10-10 | ||||||||
x10-23 | ||||||||
x10-23 | ||||||||
x10-23 | ||||||||
x10-23 | ||||||||
Cascade | x10-10 | |||||||
Cascade | x10-10 | |||||||
x10-10 | ||||||||
As an example of the notation in the column of decay modes, the sigma + decay mode is written
which implies there are two common decay modes,
Particles which decay by the strong interaction typically do so on a time scale of about 10-23 seconds, and some of the baryons listed in the table above have lifetimes on the order of 10-10 seconds. The reason for those longer lifetimes is that they are forbidden by some kind of conservation law from decaying by the strong interaction, and must decay by the weak interaction.
These decays involve quark transformations which can be represented by Feynman diagrams.There are dozens of other baryons (i.e., combinations of three quarks), but most of them decay by the strong interaction and are so short lived that they don't leave a measurable track in a bubble chamber or other detection device. These baryons have been detected as "resonances" or peaks in the probability for particle interactions.
Sem comentários:
Enviar um comentário