Quark
Nobel Prizes (5) - Gell-Man e David J. Gross, H. David Politzer and Frank Wilczek e Leon Lederman
Quark, na física de partículas, é um dos dois elementos básicos que constituem a matéria (o outro é o léptão) e é a única, entre as partículas, que interage através de todas as quatro forças fundamentais. O quark é um férmião fundamental com carga hadrónica ou cor. Não se observaram ainda quarks em estado livre. Segundo o Modelo Padrão, os quarks ocorrem em seis tipos na natureza: "top", "bottom", "charm", "strange", "up" e "down". Os dois últimos formam os prótons e nêutrons, enquanto os quatro primeiros são formados em hádrons instáveis em aceleradores de partículas.
Os quarks têm uma unidade de carga hadrônica, que aparece em três tipos distintos (cores). O campo hadrônico é também chamado de força nuclear forte. A teoria que estuda a dinâmica de quarks e das cargas hadrônicas (mediadas pelos glúons) é chamada Cromodinâmica Quântica.
Segundo a Cromodinâmica Quântica, os quarks podem formar estados ligados aos pares e às trincas. Os pares de quarks são chamados mésons e as trincas bárions. O protão é uma trinca de quarks, formado por dois quarks "up" e um quark "down". O neutrão é outro estado ligado de três quarks, dois deles "down" e um "up".
Segundo a Cromodinâmica Quântica, os quarks podem formar estados ligados aos pares e às trincas. Os pares de quarks são chamados mésons e as trincas bárions. O protão é uma trinca de quarks, formado por dois quarks "up" e um quark "down". O neutrão é outro estado ligado de três quarks, dois deles "down" e um "up".
Os quarks têm carga elétrica -1/3 ou 2/3, em que a unidade é a carga do elétron. Antipartículas dos quarks têm carga oposta. Os quarks também interagem com a força nuclear fraca, a qual transmuta tipos distintos de quarks. Por exemplo, o quark tipo "down" pode mudar para um quark tipo "up" pela emissão de um bóson vetorial massivo, que transporta a força nuclear fraca. Tal mecanismo está por trás da desintegração do neutrão.
Apesar de não serem observados em estado livre, a massa dos quarks pode ser inferida dos hádrons e mésons observados. Sabe-se que os quarks "up" e "down" tem massa comparável com a do eletrão, enquanto o quark "top" tem uma massa cerca de 200 vezes maior que a do protão.
A propriedade mais importante dos quarks é chamada de confinamento. É um fato experimental que os quarks individuais não são vistos — Eles estão sempre confinados ao interior dos hádrons, partículas subatómicas como os protões, neutrões, e méson. Esperava-se que esta propriedade fundamental surgisse da moderna teoria das interações forte, chamada de cromodinâmica quântica(QCD). Embora não exista nenhuma derivação matemática de confinamento na QCD, é fácil mostrar isto usando a teoria grade gauge.
Quarks livres
Nenhuma pesquisa para quarks livres ou carga elétrica fracionária produziu uma evidência convincente. A ausência de quarks livres foi então sendo incorporada na noção de confinamento, o qual, acredita-se, a teoria de quark deve possuir. Contudo, deve ser possível mudar o volume do confinamento pela criação de matéria quark densa ou quente. Esta nova fase da matéria QCD foi predita teoricamente e buscas experimentais por ela já foram iniciadas.
Confinamento e propriedades do quark
Cada partícula subatômica é descrita por um pequeno conjunto de números quânticos tais como spin J, paridade P, e massa m. Usualmente estas propriedades são diretamente identificadas por experimentos. Contudo, o confinamento torna impossível medir estas propriedades nos quarks. Ao invés disto, elas devem ser inferidas pela medição das propriedades das partículas compostas que são feitas de quarks. Tais inferências são mais fáceis de serem feitas adicionando números quânticos chamados de sabor (flavor).
As particulas compostas feitas de quarks e antiquarks são os hádrons. Estes incluem os mesons os quais obtêm os seus números quânticos de um quark e de um antiquark, e os baryons, os quais obtêm os seus números quânticos de três quarks. Os quarks (e os antiquarks) que contam para os números quânticos dos hádrons são chamados quark de valência. À parte destes, muitos hádrons devem conter um número indefinido de quarks, antiquarks e glúons virtual os quais contribuem para os seus números quânticos. Cada quark virtual é denominado de mar de quarks.
Sabores
Sabores
Sabor em partículas físicas |
Números quânticos sabores
Tópicos relacionados:
|
A cada quark é atribuído um número bariônico, B = 1/3, e um numero leptônico nulo L = 0. Eles tem uma carga elétrica fracionada, Q, Q = +2/3 ou Q = −1/3. Os iniciais chamaram up-type quarks, e depois, down-type quarks.
A cada quark é atribuído um isospin fraco: Tz = +1/2 para um quark "up" e Tz = −1/2 para um quark "down". Cada vez que se dobra o isospin fraco tem-se uma nova geração de quarks. Existem três gerações, e então 6 saboresde quarks — o quark up tem os sabores u, c e t, os down os d, s, b.
A cada quark é atribuído um isospin fraco: Tz = +1/2 para um quark "up" e Tz = −1/2 para um quark "down". Cada vez que se dobra o isospin fraco tem-se uma nova geração de quarks. Existem três gerações, e então 6 saboresde quarks — o quark up tem os sabores u, c e t, os down os d, s, b.
O número de gerações de quarks e léptons são iguais no modelo padrão. O número de gerações de leptons é fortemente restrito, segundo os testes experimentais feitos no LEP e CERN e pela observação da abundância de hélio no universo. A precisão da medição da meia-vida do bóson Z no LEP restringe o número de gerações a três.
Observações astronômicas da abundância de hélio produzem resultados consistentes com essa restrição. Os resultados, de uma busca direta por uma quarta geração de quarks apontam para a existência de um limite mínimo na massa dos quarks, sendo os de quarta geração os mais leves possíveis. A mais severa limitação veio da análise dos resultados do colisor Tevatron do Fermilab, e mostra que a massa da quarta geração de quark deve ser maior que 190 GeV.
Cada sabor define um número quântico que será conservado durante a interação forte, mas não na interação fraca. A alteração da magnitude do sabor na interação fraca é codificada em uma estrutura chamada matriz CKM. Esta também determina a violação CP que é permitida no modelo padrão. Os números quânticos do sabor são descritos em detalhes no artigoSabor.
Números quânticos correspondem a simetrias não-abelianas tal como a rotação. Elas requerem mais atenção na sua extração, dado que as simetrias não são aditivas. No modelo dos quarks, a construção de um mésons se dá com um quark e um antiquark; por outro lado,bárions são constituídos por três quarks. Desde que os mésons são bósons (têm spin inteiro) e bárions são férmions (têm spin semi-inteiro), o modelo dos quarks implica que os quarks são férmions. Além disto, o fato de bárions mais leves terem spin igual a -1/2 implica que cada quark pode ter spin J = 1/2. Os spins de mésons e bárions excitados são completamente consistente com estes argumentos.
Cores
Como os quarks são férmions, o princípio de exclusão de Pauli implica que os três quarks de valência devam estar em uma combinação assimétrica em um bárion. Contudo, a carga Q = 2 bárion, Δ++ (a qual é uma dos quatro isospin Iz = 3/2 bárions) pode somente ser feita de três quarks u com spins paralelos. Como esta configuração é simétrica com respeito ao intercâmbio das cargas dos quarks, isso implica que existem outros números quânticos internos que poderão então compor combinações assimétricas. A isto se dá o nome de cor, embora não tenha nada a ver com a sensação fisiológica cores. Este número quântico é a carga envolvida na Teoria Gauge chamada de Cromodinâmica Quântica (QCD).
A outra única partícula colorida é o gluon, o qual é o bóson mediador da QCD. Tal como todas as outras teorias mediadoras não-Abelianas (e diferentemente da Eletrodinâmica Quântica) os bósons mediadores interagem com os outros devido à mesma força que afeta os quarks.
A Cor é uma simetria gauge SU(3). Os quarks são dispostos na representação fundamental, 3, desde que eles se mostrem em 3 cores. Os gluons são dispostos na representação adjunta, 8 e, por conseguinte aparecem em 8 variedades. Para mais informações a este respeito, veja carga colorida.
Massa do quark
Embora se fale da massa do quark da mesma forma que se fala da massa de qualquer outra partícula, a noção da massa do quark é mais complicada pelo fato de ele não poder ser encontrado livre na natureza. Como um resultado disto, a noção da massa do quark é uma construção teórica, a qual só faz sentido quando se especifica o procedimento usado para defini-la.
Massa corrente do quark
A aproximada simetria chiral da QCD, por exemplo, permite definir a razão entre as massas dos vários quarks (para cima, para baixo e estranho) através da combinação das massas do octeto de méson escalar no modelo quark utilizando a teoria da perturbação chiral, dado

O fato de que mu ≠ 0 é importante, dado que estes não estão no problema forte CP se mu forem eliminadas. Os valores absolutos de massa foram atualmente determinados para leis de soma QCD (também conhecido como leis da somatória da função espectral) e grade QCD. A massa determinada desta maneira é conhecida como massa corrente do quark. A conexão entre diferenças de massa corrente do quark necessita de mecanismo pleno de renormalização para estas especificações.
Massa do quark de valência
Outro, antigo, método de especificar a massa do quark foi usado na fórmula de massa de Gell-Mann-Nishijima no modelo de quark, a qual relaciona a massa do hádron às massas do quark. A massa assim determinada é chamada massa do quark constituinte, e é significantemente diferente da massa corrente do quark definida acima. A massa constituinte não tem qualquer significado dinâmico.
Massa dos quarks pesados
As massas dos quarks pesados, charme e inferior são obtidas das massas de hádrons que contêm um simples quark pesado (e um antiquark leve ou dois quarks leves) e da análise do seu quarkoma. Cálculos com grade QCD usando a teoria do quark pesado influente (HQET em inglês) ou cromodinâmica quântica não relativista (NRQCD em inglês) são usadas atualmente para determinar a massa destes quarks.
O quark topo é suficientemente pesado para que a QCD de perturbação possa ser usada para determinar sua massa. Antes da sua descoberta em 1995, a melhor estimativa teórica da massa do quark topo foi obtida da análise global de testes de precisão do Modelo Padrão. O quark topo, contudo, é o único entre os quarks que decai antes que se tenha uma chance de hadronizar. Portando, sua massa tem de ser indiretamente medida através do produto de seu decaimento. Isto pode ser feito somente em um Tevatron o qual é o único acelerador de partícula com energia bastante para produzir quarks em abundância.
Antiquarks
Os números quânticos aditivos de antiquarks são iguais em magnitude e opostos em sinal àqueles dos quarks. A simetria CPT obriga-os a terem o mesmo spin e massa que seus quarks correspondentes. Testes de simetria CPT não podem ser realizados diretamente nos quarks e antiquarks devido ao confinamento, mas podem ser feito nos hádrons. A notação de antiquarks segue à da antimatéria em geral: um quark up é referido como
, e um quark anti-up é referido como
.


Sub-estrutura
Algumas evoluções do Modelo Padrão lançaram a idéia que os quarks e leptons têm subestrutura. Em outras palavras, este modelo assume que as partículas elementares do modelo padrão são de fato partículas compostas, feitas de algum outro constituinte elementar. Tais idéias estão abertas para a fase de testes experimentais, e estas teorias são severamente limitadas por falta de dados. Não há no momento atual nenhuma evidência desta sub-estrutura.
História
A noção de quarks evoluiu da classificação dos hádrons desenvolvida independentemente em 1961 por Murray Gell-Mann e Kazuhiko Nishijima, sendo atualmente denominada como modelo quark. O esquema agrupa partículas com isospin e estranheza usando simetria unitária derivada da álgebra corrente, a qual nós reconhecemos hoje como parte de simetria chiral de QCD aproximada. Esta é uma simetria de sabor SU(3) global, a qual não deve ser confundida com a simetria de calibre da QCD.
Neste esquema os mésons leves (spin-0) e os bárions (spin-½) foram agrupados em octetos, 8, com simetria de sabor. A classificação dos bárions de spin-3/2 em uma representação 10 produziu previsão de uma nova partícula, Ω−, a qual foi descoberta em 1964, levando à larga aceitação do modelo. A desejada representação 3 foi identificada como quarks.
Este esquema ficou conhecido como "modelo das oito dobras" (do inglês eightfold way) por Gell-Mann, em uma clara referência aos octetos do modelo das oito dobras do Budismo. Ele também inventou o nome quark e atribuiu-lhe a sentença “Three quarks for Muster Mark” em Finnegans Wake de James Joyce. Os resultados negativos dos experimentos de busca do quark levaram Gell-Mann a considerá-los como ficção matemática.
A análise de certas propriedade das reações de altas energias dos hádrons levou Richard Feynman a postular a existência de subestruturas nos hádrons, as quais foram chamadas de pártons (pois elas são partes dos hádrons). Uma adaptação de seções tranversais de espalhamento profundamente inelástico derivada da álgebra corrente por James Bjorken recebeu uma explicação em termos de párton. Quando adaptação de Bjorken foi verificada experimentalmente em 1969, foi imediatamente percebido que partons e quarks poderiam ser a mesma coisa. Com a prova da liberdade assintótica na QCD em 1973 por David Gross, Frank Wilczek e David Politzer (Nobel Física), esta concepção foi firmemente estabelecida.
O quark charmoso
foi postulado por Sheldon Glashow, John Iliopoulos e Luciano Maiani em 1973 para prevenir mudanças não físicas do sabor em decaimentos fracos, os quais deveriam de outra forma ocorrer segundo o modelo padrão. A descoberta em 1975 do méson que veio a ser denominado de J/ψ levou ao reconhecimento que ele seria composto de um quark charmoso e um antiquark.
foi postulado por Sheldon Glashow, John Iliopoulos e Luciano Maiani em 1973 para prevenir mudanças não físicas do sabor em decaimentos fracos, os quais deveriam de outra forma ocorrer segundo o modelo padrão. A descoberta em 1975 do méson que veio a ser denominado de J/ψ levou ao reconhecimento que ele seria composto de um quark charmoso e um antiquark.
A existência de uma terceira geração de quarks foi predita por Makoto Kobayashi e Toshihide Maskawa em 1973, que entenderam que a observação da violação da simetria CP por kaons neutros não poderia ser acomodada no modelo padrão com duas gerações de quarks. O quark inferior foi descoberto em 1980 e o quark superior em 1996 no acelerador de partículas Tevatron, do Fermilab.
Quarks
*The masses should not be taken too seriously, because the confinement of quarks implies that we cannot isolate them to measure their masses in a direct way. The masses must be implied indirectly from scattering experiments. The numbers in the table are very different from numbers previously quoted and are based on the July 2010 summary in Journal of Physics G, Review of Particle Physics, Particle Data Group. A summary can be found on the LBL site.
These masses represent a strong departure from earlier approaches which treated the masses for the U and D as about 1/3 the mass of a proton, since in the quark model the proton has three quarks. The masses quoted are model dependent, and the mass of the bottom quark is quoted for two different models. But in other combinations they contribute different masses. In thepion, an up and an anti-down quark yield a particle of only 139.6 MeV of mass energy, while in the rho vector meson the same combination of quarks has a mass of 770 MeV! The masses of C and S are from Serway, and the T and B masses are from descriptions of the experiments in which they were discovered.
Each of the six "flavors" of quarks can have three different "colors". The quark forces are attractive only in "colorless" combinations of three quarks (baryons), quark-antiquark pairs (mesons) and possibly larger combinations such as the pentaquark that could also meet the colorless condition. Quarks undergo transformations by the exchange of W bosons, and those transformations determine the rate and nature of the decay of hadrons by the weak interaction.
Why "Quark"?
The name "quark" was taken by Murray Gell-Mann from the book "Finnegan's Wake" by James Joyce. The line "Three quarks for Muster Mark..." appears in the fanciful book. Gell-Mann received the 1969 Nobel Prize for his work in classifying elementary particles.
Up (U ) and Down ( D ) Quarks
Quarks and Leptons are the building blocks which build up matter, i.e., they are seen as the "elementary particles". In the present standard model, there are six "flavors" of quarks. They can successfully account for all known mesons and baryons (over 200). The most familiar baryons are the proton and neutron, which are each constructed from up and down quarks. Quarks are observed to occur only in combinations of two quarks (mesons), three quarks (baryons). There was a recent claim of observation of particles with five quarks (pentaquark), but further experimentation has not borne it out.
Quark | Symbol | Spin | Charge | Baryon Number | S | C | B | T | Mass* |
4.67 GeV(1S) |
*The masses should not be taken too seriously, because the confinement of quarks implies that we cannot isolate them to measure their masses in a direct way. The masses must be implied indirectly from scattering experiments. The numbers in the table are very different from numbers previously quoted and are based on the July 2010 summary in Journal of Physics G, Review of Particle Physics, Particle Data Group. A summary can be found on the LBL site.
These masses represent a strong departure from earlier approaches which treated the masses for the U and D as about 1/3 the mass of a proton, since in the quark model the proton has three quarks. The masses quoted are model dependent, and the mass of the bottom quark is quoted for two different models. But in other combinations they contribute different masses. In thepion, an up and an anti-down quark yield a particle of only 139.6 MeV of mass energy, while in the rho vector meson the same combination of quarks has a mass of 770 MeV! The masses of C and S are from Serway, and the T and B masses are from descriptions of the experiments in which they were discovered.
Each of the six "flavors" of quarks can have three different "colors". The quark forces are attractive only in "colorless" combinations of three quarks (baryons), quark-antiquark pairs (mesons) and possibly larger combinations such as the pentaquark that could also meet the colorless condition. Quarks undergo transformations by the exchange of W bosons, and those transformations determine the rate and nature of the decay of hadrons by the weak interaction.
Why "Quark"?
The name "quark" was taken by Murray Gell-Mann from the book "Finnegan's Wake" by James Joyce. The line "Three quarks for Muster Mark..." appears in the fanciful book. Gell-Mann received the 1969 Nobel Prize for his work in classifying elementary particles.
Up (U ) and Down ( D ) Quarks
The up and down quarks are the most common and least massive quarks, being the constituents of protons and neutrons and thus of most ordinary matter.
![]() | The fact that the free neutron decays![]() and nuclei decay by beta decay in processes like ![]() ![]() |
The Strange Quark ( S )
In 1947 during a study of cosmic ray interactions, a product of a proton collision with a nucleus was found to live for much longer time than expected: 10-10 seconds instead of the expected 10-23 seconds! This particle was named the lambda particle (Λ0) and the property which caused it to live so long was dubbed "strangeness" and that name stuck to be the name of one of the quarks from which the lambda particle is constructed. The lambda is a baryon which is made up of three quarks: an up, a down and a strange quark.
The shorter lifetime of 10-23 seconds was expected because the lambda as a baryon participates in the strong interaction, and that usually leads to such very short lifetimes. The long observed lifetime helped develop a new conservation law for such decays called the "conservation of strangeness". The presence of a strange quark in a particle is denoted by a quantum number S=-1. Particle decay by the strong or electromagnetic interactions preserve the strangeness quantum number.
The decay process for the lambda particle must violate that rule, since there is no lighter particle which contains a strange quark - so the strange quark must be transformed to another quark in the process. That can only occur by the weak interaction, and that leads to a much longer lifetime. The decay processes show that strangeness is not conserved:
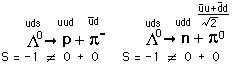
The quark transformations necessary to accomplish these decay processes can be visualized with the help of Feynmann diagrams.
![]() | The omega-minus, a baryon composed of three strange quarks, is a classic example of the need for the property called "color" in describing particles. Since quarks are fermions with spin 1/2, they must obey the Pauli exclusion principle and cannot exist in identical states. So with three strange quarks, the property which distinguishes them must be capable of at least three distinct values. |
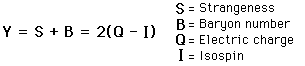
Isospin and either hypercharge or strangeness are the quantum numbers often used to draw particle diagrams for the hadrons.
The Charm Quark ( C )
In 1974 a meson called the J/Psi particle was discovered. With a mass of 3100 MeV, over three times that of the proton, this particle was the first example of another quark, called the charm quark. The J/Psi is made up of a charm-anticharm quark pair.
The lightest meson which contains a charm quark is the D meson. It provides interesting examples of decay since the charm quark must be transformed into a strange quark by the weak interaction in order for it to decay.
The lightest meson which contains a charm quark is the D meson. It provides interesting examples of decay since the charm quark must be transformed into a strange quark by the weak interaction in order for it to decay.
One baryon with a charm quark is a called a lambda with symbol Λ+c . It has a composition udc and a mass of 2281 MeV/c2.
The Top Quark ( T )
Convincing evidence for the observation of the top quark was reported by Fermilab 's Tevatron facility in April 1995. The evidence was found in the collision products of 0.9 TeV protons with equally energetic antiprotons in the proton-antiproton collider. The evidence involved analysis of trillions of 1.8 TeV proton-antiproton collisions. The Collider Detector Facility group had found 56 top candidates over a predicted background of 23 and the D0 group found 17 events over a predicted background of 3.8.
The value for the top quark mass from the combined data of the two groups after the completion of the run was 174.3 +/- 5.1 GeV. This is over 180 times the mass of a proton and about twice the mass of the next heaviest fundamental particle, the Z0 vector boson at about 93 GeV. The interaction is envisioned as follows:
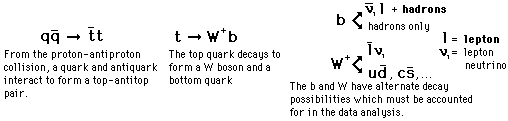
Confinement of Quarks
How can one be so confident of the quark model when no one has ever seen an isolated quark? There are good reasons for the lack of direct observation. Apparently the color force does not drop off with distance like the other observed forces. It is postutated that it may actually increase with distance at the rate of about 1 GeV per fermi.
A free quark is not observed because by the time the separation is on an observable scale, the energy is far above the pair production energy for quark-antiquark pairs. For the U and D quarks the masses are 10s of MeV so pair production would occur for distances much less than a fermi. You would expect a lot of mesons (quark-antiquark pairs) in very high energy collision experiments and that is what is observed.
Basically, you can't see an isolated quark because the color force does not let them go, and the energy required to separate them produces quark-antiquark pairs long before they are far enough apart to observe separately.
One kind of visualization of quark confinement is called the "bag model". One visualizes the quarks as contained in an elastic bag which allows the quarks to move freely around, as long as you don't try to pull them further apart. But if you try to pull a quark out, the bag stretches and resists.
Another way of looking at quark confinement is expressed by Rohlf. "When we try to pull a quark out of a proton, for example by striking the quark with another energetic particle, the quark experiences a potential energy barrier from the strong interaction that increases with distance." As the example of alpha decay demonstrates, having a barrier higher than the particle energy does not prevent the escape of the particle - quantum mechanical tunneling gives a finite probability for a 6 MeV alpha particle to get through a 30 MeV high energy barrier. But the energy barrier for the alpha particle is thin enough for tunneling to be effective. In the case of the barrier facing the quark, the energy barrier does not drop off with distance, but in fact increases.
The Bottom Quark ( B )
In 1977, an experimental group at Fermilab led by Leon Lederman discovered a new resonance at 9.4 GeV/c^2 which was interpreted as a bottom-antibottom quark pair and called the Upsilon meson. From this experiment, the mass of the bottom quark is implied to be about 5 GeV/c^2. The reaction being studied was

where N was a copper or platinum nucleus. The spectrometer had a muon-pair mass resolution of about 2%, which allowed them to measure an excess of events at 9.4 GeV/c^2. This resonance has been subsequently studied at other accelerators with a detailed investigation of the bound states of the bottom-antibottom meson.
Pentaquark
In 2003 came experimental evidence of a five-quark combination which is being called a pentaquark. Strong evidence for the pentaquark came from experiments at the Jefferson Lab in Newport News, Virginia during 2003. The experiments involved multi-GeV photons impacting a deuterium target. The evidence showed a five-quark baryon state at a mass of 1.54 GeV with a narrow width of 22 MeV.
The new particle is being called Theta-plus, with a composition of two up quarks, two down quarks and an anti-strange quark. The combination of quark charges +2/3(u), -1/3 (d) and 1/3 (anti-strange) gives a net charge of +1. The lifetime of the particle is about 10-20 seconds. The decay is classified as a strong interaction decay to a neutron and a K+ meson
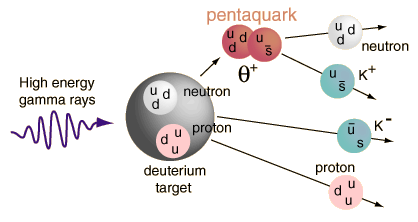
The Jefferson Lab results corroborated the growing body of evidence for the pentaquark from the last few years. Work done by a Japanese team led by Takashi Nakano of Osaka University involved bombarding a carbon target with X-rays and led to a report of pentaquark evidence in 2002. A retrospective study of 1986 bubble chamber experiments at ITEP in Moscow also revealed a 1.54 GeV peak. A review of data taken in 1997/98 at the ELSA accelerator in Bonn, Germany showed evidence of a particle of mass 1.54 GeV and width less than 25 MeV. The existence of the pentaquark was hypothesized by Maxim Polyakov, Dmitri Diakonov, and Victor Petrov at the Petersburg Nuclear Physics Institute in Russia in 1997.
While the postulated pentaquark has made an interesting story, continued experimentation has not borne it out. The 2006 Particle Data Group publication expressed its pessimism with the statement :
"There has not been a high-statistics confirmation of any of the original experiments that claimed to see the θ+; there have been two high-statistics repeats from Jefferson Lab that have clearly shown the original positive claims in those two cases to be wrong; there have been a number of other high-statistics experiments, none of which have found any evidence for the θ+; and all attempts to confirm the two other claimed pentaquark states have led to negative results. The conclusion that pentaquarks in general, and the θ+, in particular, do not exist, appears compelling."
The statement in the 2008 Summary was even stronger, and the issue appears to have been dropped from the 2010 Summary, so the consensus seems strong that this particular quark combination has not been observed.
Mesons
Mesons are intermediate mass particles which are made up of a quark-antiquark pair. Three quark combinations are called baryons. Mesons are bosons, while the baryons are fermions. Recent experimental evidence shows the existence of five-quark combinations which are being called pentaquarks.
pion
Particle | Symbol | Anti- particle | Makeup | MeV/c2 | S | C | B | Lifetime | |
0 | x10-8 | ||||||||
![]() | 0 | x10-16 |
The neutral pion decays to an electron, positron, and gamma ray by the electromagnetic interaction on a time scale of about 10-16 seconds. The positive and negative pions have longer lifetimes of about 2.6 x 10-8 s.
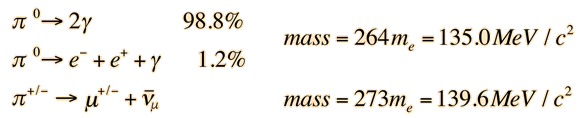
The negative pion decays into a muon and a muon antineutrino as illustrated below. This decay is puzzling upon first examination because the decay into an electron plus an electron antineutrino yields much more energy. Usually the pathway with the greatest energy yield is the preferred pathway. This suggests that some symmetry is acting to inhibit the electron decay pathway.
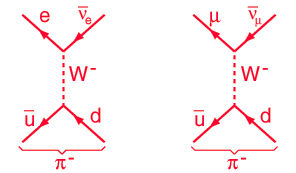
The symmetry which suppresses the electron pathway is that of angular momentum, as described by Griffiths. Since the negative pion has spin zero, the electron and antineutrino must be emitted with opposite spins to preserve net zero spin. But the antineutrino is always right-handed, so this implies that the electron must be emitted with spin in the direction of its linear momentum (i.e., also right-handed).
But if the electron were massless, it would (like the neutrino) only exist as a left-handed particle, and the electron pathway would be completely prohibited. So the suppression of the electron pathway is attributed to the fact that the electron's small mass greatly favors the left-handed symmetry, thus inhibiting the decay. Weak interaction theory predicts that the fraction of muons decaying into electrons should be 1.28 x 10-4 and the measured branching ratio is 1.23 +/- 0.02 x 10-4.
The pion, being the lightest meson, can be used to predict the maximum range of the strong interaction. The strong interaction properties of the three pions are identical. The connection between pions and the strong force was proposed by Hideki Yukawa. Yukawa worked out a potential for the force and predicted its mass based on the uncertainty principle from measurements of the apparent range of the strong force in nuclei.
Being composed of an up and an antidown quark, the positive pion would be expected to have a mass about 2/3 that of a proton, yet it's mass is only about 1/6 of that of the proton! This is an example of how hadron masses depend upon the dynamics inside the particle, and not just upon the quarks contained.
The pion is a meson. The π+ is considered to be made up of an up and an anti-down quark. The neutral pion is considered to be a combination
![]() | ![]() |
Pions interact with nuclei and transform a neutron to a proton or vice versa:

The pions π+ and π- have spin zero and negative intrinsic parity (Rohlf Sec 17-2).
The psi/J Particle
Particle | Symbol | Anti- particle | Makeup | MeV/c2 | S | C | B | Lifetime | |
0 | x10-20 |
The psi/J particle is a meson which was discovered in 1974 by experimenters at Stanford (Richter) and Brookhaven National Laboratory (Ting). Slightly more than three times as massive as the proton, this particle decayed slowly and didn't fit into the framework of the up, down, and strange quarks. It is considered to be a charm-anticharm quark pair and was the first firm experimental evidence for the fourth quark. Richter and Ting shared the 1976 Nobel Prize for their discovery.
Particle | Symbol | Anti- particle | Makeup | MeV/c2 | S | C | B | Lifetime | |
0 | x10-20 |
The upsilon particle is a meson which was discovered at Fermilab in 1977. It appeared as another long-lived particle which didn't fit into the framework of the first four quarks, the up,down, strange, and charm quarks. It is taken as a botton-antibottom quark pair and was the first experimental evidence of the fifth quark.
Particles that interact by the strong interaction are called hadrons. This general classification includes mesons and baryons but specifically excludes leptons, which do not interact by the strong force. The weak interaction acts on both hadrons and leptons.
Hadrons are viewed as being composed of quarks, either as quark-antiquark pairs (mesons) or as three quarks (baryons). There is much more to the picture than this, however, because the constituent quarks are surrounded by a cloud of gluons, the exchange particles for the color force.
Recent experimental evidence shows the existence of five-quark combinations which are being called pentaquarks.
Hadrons are viewed as being composed of quarks, either as quark-antiquark pairs (mesons) or as three quarks (baryons). There is much more to the picture than this, however, because the constituent quarks are surrounded by a cloud of gluons, the exchange particles for the color force.
Recent experimental evidence shows the existence of five-quark combinations which are being called pentaquarks.
Baryons (Proton and neutron )
Baryons are massive particles which are made up of three quarks in the standard model. This class of particles includes the proton and neutron. Other baryons are the lambda, sigma, xi, and omega particles. Baryons are distinct from mesons in that mesons are composed of only two quarks. Baryons and mesons are included in the overall class known as hadrons, the particles which interact by the strong force. Baryons are fermions, while the mesons are bosons. Besides charge and spin (1/2 for the baryons), two other quantum numbers are assigned to these particles: baryon number (B=1) and strangeness (S), which in the chart can be seen to be equal to -1 times the number of strange quarks included.
The conservation of baryon number is an important rule for interactions and decays of baryons. No known interactions violate conservation of baryon number. Recent experimental evidence shows the existence of five-quark combinations which are being called pentaquarks. The pentaquark would be included in the classification of baryons, albeit an "exotic" one. The pentaquark is composed of four quarks and an antiquark, like a combination of an ordinary baryon plus a meson.
Table of Baryons
Particle | Symbol | Makeup | Rest mass MeV/c2 | Spin | B | S | (seconds> | |
x10-10 | ||||||||
x10-10 | ||||||||
x10-10 | ||||||||
x10-23 | ||||||||
x10-23 | ||||||||
x10-23 | ||||||||
x10-23 | ||||||||
Cascade | x10-10 | |||||||
Cascade | x10-10 | |||||||
x10-10 | ||||||||
which implies there are two common decay modes,
Particles which decay by the strong interaction typically do so on a time scale of about 10-23 seconds, and some of the baryons listed in the table above have lifetimes on the order of 10-10 seconds. The reason for those longer lifetimes is that they are forbidden by some kind of conservation law from decaying by the strong interaction, and must decay by the weak interaction.
These decays involve quark transformations which can be represented by Feynman diagrams.There are dozens of other baryons (i.e., combinations of three quarks), but most of them decay by the strong interaction and are so short lived that they don't leave a measurable track in a bubble chamber or other detection device. These baryons have been detected as "resonances" or peaks in the probability for particle interactions.
Mesons
Mesons are intermediate mass particles which are made up of a quark-antiquark pair. Three quark combinations are called baryons. Mesons are bosons, while the baryons are fermions.

Particle | Symbol | Anti- particle | Makeup | MeV/c2 | S | C | B | Lifetime | |
0 | x10-8 | ||||||||
![]() | 0 | x10-16 | |||||||
0 | x10-8 | ||||||||
0 | x10-10 | ||||||||
0 | x10-8 | ||||||||
0 | |||||||||
0 | |||||||||
0 | x10-23 | ||||||||
0 | x10-23 | ||||||||
0 | x10-22 | ||||||||
0 | x10-23 | ||||||||
0 | x10-13 | ||||||||
0 | x10-13 | ||||||||
0 | x10-13 | ||||||||
0 | x10-20 | ||||||||
-1 | x10-12 | ||||||||
-1 | x10-12 | ||||||||
-1 | |||||||||
0 | x10-20 |
1* The neutral Kaons K0s and K0L represent symmetric and antisymmetric mixtures of the quark combinations down-antistrange and antidown-strange.
2* The neutral eta meson is considered to be a quark combination
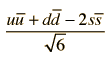
Note: In the table, antiquarks are indicated by an underline, e.g., ud means a combination of an up and an antidown quark.
Most of the listed decay times are in the range 10-13 to 10-8 s which indicates decay by the weak interaction. These decays involve quark transformations which can be represented by Feynman diagrams.
Leptons and quarks are the basic building blocks of matter, i.e., they are seen as the "elementary particles". There are six leptons in the present structure, the electron, muon, and tau particles and their associated neutrinos. The different varieties of the elementary particles are commonly called "flavors", and the neutrinos here are considered to have distinctly different flavor.
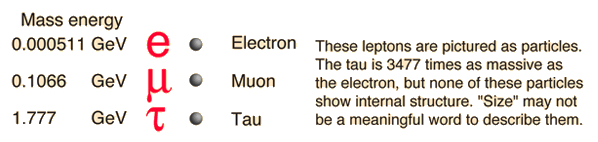
Important principles for all particle interactions are the conservation of lepton number and the the conservation of baryon number.
Now that we have experimental evidence for six leptons, a relevant question is "Are there more?". The present standard model assumes that there are no more than three generations. One of the pieces of experimental evidence for that is the measured hydrogen/helium abundance ratio in the universe. When the process of nucleosynthesis from the big bang is modeled, the number of types of neutrinos affects the abundance of helium. The observed abundance agrees with three types of neutrinos.
Electron and Positron ( Are leptons )
As one of the leptons, the electron is viewed as one of the fundamental particles. It is a fermion of spin 1/2 and therefore constrained by the Pauli exclusion principle, a fact that has key implications for the building up of the periodic table of elements.
The electron's antiparticle, the positron, is identical in mass but has a positive charge. If an electron and a positron encounter each other,they will annihilate with the production of two gamma-rays. On the other hand, one of the mechanisms for the interaction of radiation with matter is the pair production of an electron-positron pair. Associated with the electron is a the electron neutrino
.
Particle | Symbol | Anti- particle | MeV/c2 | L(e) | L(muon) | L(tau) | (seconds) |
(Electron) |
Muon
The muon is a lepton which decays to form an electron or positron.
The fact that the above decay is a three-particle decay is an example of the conservation of lepton number; there must be one electron neutrino and one muon neutrino or antineutrino in the decay.
The lifetime of the muon is 2.20 microseconds. The muon is produced in the upper atmosphere by the decay of pions produced by cosmic rays:

Measuring the flux of muons of cosmic ray origin at different heights above the earth is an important time dilation experiment in relativity.
Muons make up more than half of the cosmic radiation at sea level, the remainder being mostly electrons, positrons and photons from cascade events.(Richtmyer) The average sea level muon flux is about 1 muon per square centimeter per minute.
Particle | Symbol | Anti- particle | MeV/c2 | L(e) | L(muon) | L(tau) | (seconds) |
(Muon) |
Tau
The tau is the most massive of the leptons, having a rest mass some 3490 times the mass of the electron, also a lepton. Its mass is some 17 times that of the muon, the other massive lepton.
Particle | Symbol | Anti- particle | MeV/c2 | L(e) | L(muon) | L(tau) | (seconds) |
(Tau) |
Sem comentários:
Enviar um comentário