Nuclear Medicine
There are a number of processes involved in health care that make use of the properties of the nucleus. Nuclear medicine is a broad term encompassing both diagnostic and therapeutic processes. To some extent this is unfortunate, since in the cases where nuclear radiation is involved the doses involved in diagnostic procedures are very small and those in therapeutic applications are very large.
It is therefore important to make clear distinctions between the risks involved in diagnostic and therapeutic applications. Still another class of processes include diagnostic procedures such as magnetic resonance imaging which involve nuclear properties but do not involve any exposure to ionizing radiation.
Nuclear medicine is a medical specialty involving the application of radioactive substances in the diagnosis and treatment of disease.
In nuclear medicine procedures, radionuclides are combined with other elements to form chemical compounds, or else combined with existing pharmaceutical compounds, to form radiopharmaceuticals. These radiopharmaceuticals, once administered to the patient, can localize to specific organs or cellular receptors. This property of radiopharmaceuticals allows nuclear medicine the ability to image the extent of a disease process in the body, based on the cellular function and physiology, rather than relying on physical changes in the tissue anatomy.
In some diseases, nuclear medicine studies can identify medical problems at an earlier stage than other diagnostic tests. Nuclear medicine, in a sense, is "radiology done inside out", or "endo-radiology", because it records radiation emitting from within the body rather than radiation that is generated by external sources like X-rays.
Treatment of diseased tissue, based on metabolism or uptake or binding of a particular ligand, may also be accomplished, similar to other areas of pharmacology. However, the treatment effects of radiopharmaceuticals rely on the tissue-destructive power of short-range ionizing radiation.
In the future, nuclear medicine may provide added impetus to the field known as molecular medicine. As understanding of biological processes in the cells of living organisms expands, specific probes can be developed to allow visualization, characterization, and quantification of biologic processes at the cellular and subcellular levels. Nuclear medicine is a possible specialty for adapting to the new discipline of molecular medicine because of its emphasis on function and its utilization of imaging agents that are specific for a particular disease process.
Diagnostic sonography (ultrasonography)
Diagnostic sonography (ultrasonography) is an ultrasound-based diagnostic imaging technique used for visualizing subcutaneous body structures including tendons, muscles, joints, vessels and internal organs for possible pathology or lesions. Obstetric sonographyis commonly used during pregnancy.
In physics, the term "ultrasound" applies to all sound waves with a frequency above the audible range of normal human hearing, about 20 kHz. The frequencies used in diagnostic ultrasound are typically between 2 and 18 MHz.
Typical diagnostic sonographic scanners operate in the frequency range of 2 to 18 megahertz, though frequencies up to 50–100 megahertz have been used experimentally in a technique known as biomicroscopy in special regions, such as the anterior chamber of the eye. The choice of frequency is a trade-off between spatial resolution of the image and imaging depth: lower frequencies produce less resolution but image deeper into the body.
Higher frequency sound waves have a smaller wavelength and thus are capable of reflecting or scattering from smaller structures. Higher frequency sound waves also have a larger attenuation coefficient and thus are more readily absorbed in tissue, limiting the depth of penetration of the sound wave into the body.
Sonography (ultrasonography) is widely used in medicine. It is possible to perform both diagnosis and therapeutic procedures, using ultrasound to guide interventional procedures (for instance biopsies or drainage of fluid collections). Sonographers are medical professionals who perform scans which are then typically interpreted by radiologists, physicians who specialize in the application and interpretation of a wide variety of medical imaging modalities, or by cardiologists in the case of cardiac ultrasonography (echocardiography).
Producing a sound wave
A sound wave is typically produced by a piezoelectric transducer encased in a housing which can take a number of forms. Strong, short electrical pulses from the ultrasound machine make the transducer ring at the desired frequency. The frequencies can be anywhere between 2 and 18 MHz.
The sound is focused either by the shape of the transducer, a lens in front of the transducer, or a complex set of control pulses from the ultrasound scanner machine (Beam forming). This focusing produces an arc-shaped sound wave from the face of the transducer. The wave travels into the body and comes into focus at a desired depth.
Older technology transducers focus their beam with physical lenses. Newer technology transducers use phased array techniques to enable the sonographic machine to change the direction and depth of focus. Almost all piezoelectric transducers are made of ceramic.
Materials on the face of the transducer enable the sound to be transmitted efficiently into the body (usually seeming to be a rubbery coating, a form of impedance matching). In addition, a water-based gel is placed between the patient's skin and the probe.
The sound wave is partially reflected from the layers between different tissues. Specifically, sound is reflected anywhere there are density changes in the body: e.g. blood cells in blood plasma, small structures in organs, etc. Some of the reflections return to the transducer.
Receiving the echoes
The return of the sound wave to the transducer results in the same process that it took to send the sound wave, except in reverse. The return sound wave vibrates the transducer, the transducer turns the vibrations into electrical pulses that travel to the ultrasonic scanner where they are processed and transformed into a digital image.
The frequencies used for medical imaging are generally in the range of 1 to 18 MHz. Higher frequencies have a correspondingly smaller wavelength, and can be used to make sonograms with smaller details. However, the attenuation of the sound wave is increased at higher frequencies, so in order to have better penetration of deeper tissues, a lower frequency (3–5 MHz) is used.
Seeing deep into the body with sonography is very difficult. Some acoustic energy is lost every time an
echo is formed, but most of it (approximately
) is lost from acoustic absorption. (See also Acoustic attenuation for further details on modeling of acoustic attenuation and absorption.)

Ultrasonic Transducers
Ultrasonic sound can be produced by transducers which operate either by the piezoelectric effect or the magnetostrictive effect. The magnetostrictive transducers can be used to produce high intensity ultrasonic sound in the 20-40 kHz range for ultrasonic cleaning and other mechanical applications.
Ultrasonic medical imaging typically uses much higher ultrasound frequencies in the range 1-20 MHz. Such ultrasound is produced by applying the output of an electronic oscillator to a thin wafer of piezoelectric material such as lead zirconate titanate. The higher frequencies imply shorter wavelengths and therefore higher resolution for the imaging process.
The application of the basic ideas of imaging (e.g., the Rayleigh criterion) suggests that the resolution of any imaging process is limited by diffraction to a dimension similar to the wavelength of the wave used for the imaging process.
Piezoelectric Effect
Crystals which acquire a charge when compressed, twisted or distorted are said to be piezoelectric. This provides a convenient transducer effect between electrical and mechanical oscillations. Quartz demonstrates this property and is extremely stable. Quartz crystals are used for watch crystals and for precise frequency reference crystals for radio transmitters. Rochelle salt produces a comparatively large voltage upon compression and was used in early crystal microphones.
Barium titanate, lead zirconate, and lead titanate are ceramic materials which exhibit piezoelectricity and are used in ultrasonic transducers as well as microphones. If an electrical oscillation is applied to such ceramic wafers, they will respond with mechanical vibrations which provide the ultrasonic sound source. The standard piezoelectric material for medical imaging processes has been lead zirconate titanate (PZT). Piezoelectric ceramic materials have found use in producing motions on the order of nanometers in the control of scanning tunneling microscopes.
The word piezo is Greek for "push". The effect known as piezoelectricity was discovered by brothers Pierre and Jacques Curie when they were 21 and 24 years old in 1880.
There is a magnetic analog where ferromagnetic material respond mechanically to magnetic fields. This effect, called magnetostriction, is responsible for the familiar hum of transformers and other AC devices containing iron cores.
Scanning Tunneling Microscope
The subject of a 1986 Nobel Prize, the scanning tunneling microscope is currently the most powerful and versatile tool for imaging individual atoms. If a pointed metal probe is placed sufficiently close to a solid sample and a voltage of say 10 millivolts is applied between the probe and the surface, then electron tunneling can occur.
The separation between the mini-tip of the probe and the sample must be on the order of nanometers. The exponential variation of the tunneling current with separation can show surface variations in the range 0.01 nanometers. The sharpening of the mini-tip of the probe is accomplished with electrochemical etching. This etching sharpens it to only about 1000 nm, but since the sharpening is not perfectly smooth, it leaves a surface with many mini-tips which are on the scale of nanometers.
Positioning a probe over the nanometer range can be done with piezoelectric ceramic wafers.
Gert Binnig and Heinrich Rohrer were awarded the Nobel Prize in 1986 along with Ernst Ruska for his work in developing electron microscopy.
Doppler echocardiography
Is a procedure which uses ultrasound technology which can examine the heart or blood vessels. An echocardiogram uses high frequency sound waves to create an image of the heart while the use of Doppler technology allows determination the speed and direction of blood flow by utilizing the Doppler effect.
An echocardiogram can, within certain limits, produce accurate assessment of the direction of blood flow and the velocity of blood and cardiac tissue at any arbitrary point using the Doppler effect. One of the limitations is that the ultrasound beam should be as parallel to the blood flow as possible.
Velocity measurements allow assessment of cardiac valve areas and function, any abnormal communications between the left and right side of the heart, any leaking of blood through the valves (valvular regurgitation), calculation of the cardiac output and calculation of E/A ratio (a measure of diastolic dysfunction). Contrast-enhanced ultrasound using gas-filled microbubble contrast media can be used to improve velocity or other flow-related medical measurements.
An advantage of Doppler echocardiography is that it can be used to measure blood flow within the heart without invasive procedures such as cardiac catheterisation. For example, left ventricular filling pressure can be estimated by Doppler ultrasound, although only under certain conditions.
X-ray computed tomography, also computed tomography (CT scan), computed axial tomography (CAT scan) or computer assisted tomography is a medical imagingprocedure that uses computer-processed X-rays to produce tomographic images or 'slices' of specific areas of the body. These cross-sectional images are used for diagnostic and therapeutic purposes in various medical disciplines.
Digital geometry processing is used to generate a three-dimensional image of the inside of an object from a large series of two-dimensional X-ray images taken around a single axis of rotation.
![]() |
A historic EMI-Scanner |
The first commercially viable CT scanner was invented by Sir Godfrey Hounsfield (Medicine Nobel Prize in 1979) in Hayes, United Kingdom, at EMI Central Research Laboratories using X-rays. Hounsfield conceived his idea in 1967. The first EMI-Scanner was installed in Atkinson Morley Hospitalin Wimbledon, England, and the first patient brain-scan was done on 1 October 1971.[66] It was publicly announced in 1972.
The original 1971 prototype took 160 parallel readings through 180 angles, each 1° apart, with each scan taking a little over 5 minutes. The images from these scans took 2.5 hours to be processed by algebraic reconstruction techniques on a large computer. The scanner had a single photomultiplier detector, and operated on the Translate/Rotate principle.
Magnetic Resonance Imaging
Proton nuclear magnetic resonance (NMR) detects the presence of hydrogens (protons) by subjecting them to a large magnetic field to partially polarize the nuclear spins, then exciting the spins with properly tuned radio frequency (RF) radiation, and then detecting weak radio frequency radiation from them as they "relax" from this magnetic interaction. The frequency of this proton "signal" is proportional to the magnetic field to which they are subjected during this relaxation process.
In the medical application known as Magnetic Resonance Imaging (MRI), an image of a cross-section of tissue can be made by producing a well-calibrated magnetic field gradient across the tissue so that a certain value of magnetic field can be associated with a given location in the tissue. Since the proton signal frequency is proportional to that magnetic field, a given proton signal frequency can be assigned to a location in the tissue.
This provides the information to map the tissue in terms of the protons present there. Since the proton density varies with the type of tissue, a certain amount of contrast is achieved to image the organs and other tissue variations in the subject tissue.
![]() |
Since the MRI uses proton NMR, it images the concentration of protons. Many of those protons are the protons in water, so MRI is particularly well suited for the imaging of soft tissue, like the brain, eyes, and other soft tissue structures in the head as shown at left. The bone of the skull doesn't have many protons, so it shows up dark. Also the sinus cavities image as a dark region.
Bushong's assessment is that about 80% of the body's atoms are hydrogen atoms, so most parts of the body have an abundance of sources for the hydrogen NMR signals which make up the magnetic resonance image.
|
The schematic below may help visualize the imaging process. It is presumed that there are two regions of the sample which contain enough hydrogens to produce a strong NMR signal. The top sketch visualizes an NMR process with a constant magnetic field applied to the entire sample. The hydrogen spin-flip frequency is then the same for all parts of the sample.
Once excited by the RF signal, the hydrogens will tend to return to their lower state in a process called "relaxation" and will re-emit RF radiation at their Larmor frequency. This signal is detected as a function of time, and then is converted to signal strength as a function of frequency by means of a Fourier transformation.
Since the protons in each of the active areas of the sample are subjected to the same magnetic field, they will produce the same frequency of radiation and the Fourier transform of the detected signal will have only one peak. This one peak demonstrates the presence of hydrogen atoms, but gives no information to locate them in the sample.
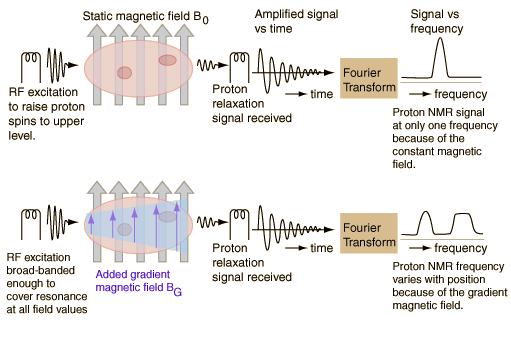
Information about the location of the hydrogen atoms can be obtained by adding a calibrated gradient field across the region of the sample as shown in the bottom sketch above. With an increasing magnetic field as you move to the right across the sample, the spin-flip energy and therefore the frequency of the emitted signal increases from left to right.
When excited by an RF transmitter, the emitted signal contains different frequencies for the two proton concentration areas. These frequencies can be separated by means of the Fourier transform and the example gives two different regions of frequency for the two sample areas. This is the beginning of the process of locating the hydrogen atoms. In the sketch, it only locates them along the horizontal direction, giving no indication that they are at different heights.
![]() |
When a rotating field gradient is used, linear positioning information is collected along a number of different directions. That information can be combined to produce a two-dimensional map of the proton densities.
The proton NMR signals are quite sensitive to differences in proton content that are characteristic of different kinds of tissue. Even though the spatial resolution of MRI is not as great as a conventional x-ray film, its contrast resolution is much better for tissue. Rapid scanning and computer reconstruction give well-resolved images of organs.
|
Myocardial Infusion Imaging
A widely used diagnostic procedure for heart health is the imaging of the blood infusion to the heart using a low dose of a radioactive tracer in the blood. A large radiation detector rotates about the chest of the patient, detecting gamma radiation from the tracer element which has been injected into the patient's vein. The image shows the perfusion of the blood into the heart muscle.
![]() |
The detection of the gamma rays produces images of the blood flow in the heart muscle from various angles to provide an assessment of the infusion of blood
Early tests of this type used an isotope of thallium with a gamma ray of energy about 70 keV. This kind of image came to be known as a "thallium scan", but now an isotope of technetium is the isotope of choice for the scans, having a shorter halflife and producing a gamma of energy about 140 keV.
The large detector uses an ionization detection in a manner similar to a Geiger counter and collects a series of exposures as it sequences around the body trunk. A collection of images is illustrated below, taken before and after exercise on a treadmill for comparison of the infusion of the blood under those two conditions.
|
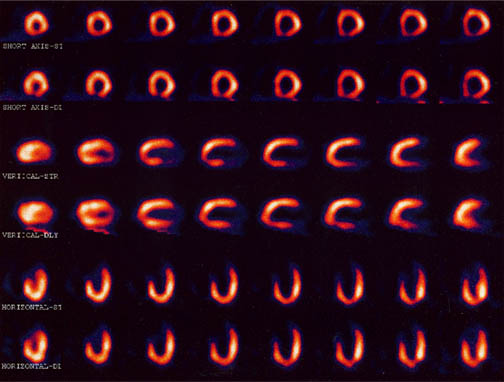
PET Scan
An interesting application in nuclear medicine is the use of positron annihilation in positron emission tomography or PET. Certain radioisotopes decay by positron emission, and such radioisotopes can be used as tracers. If injected into the body, they can be readily followed because the emission of the annihilation pairs of coincident gamma rays at 180¡ allows their source to be located along a line. Data collection for emissions at several angles permits precise location of any concentration of the radioisotope.
Positron emission tomography (PET) is a nuclear medical imaging technique that produces a three-dimensional image or picture of functional processes in the body. The system detects pairs of gamma rays emitted indirectly by a positron-emitting radionuclide(tracer), which is introduced into the body on a biologically active molecule. Three-dimensional images of tracer concentration within the body are then constructed by computer analysis. In modern scanners, three dimensional imaging is often accomplished with the aid of a CT X-ray scan performed on the patient during the same session, in the same machine.
If the biologically active molecule chosen for PET is FDG, an analogue of glucose, the concentrations of tracer imaged will indicate tissue metabolic activity by virtue of the regional glucose uptake. Use of this tracer to explore the possibility of cancer metastasis (i.e., spreading to other sites) is the most common type of PET scan in standard medical care (90% of current scans). However, on a minority basis, many other radiotracers are used in PET to image the tissue concentration of many other types of molecules of interest.
An image of a slice of the body (called a tomograph) can be constructed by using a ring of detectors. When a positron is emitted by a nucleus, it almost instantly finds an electron and the pair annihilates, converting all the mass energy of the two particles into two gamma rays. The two gamma ray photons possess momentum, and theconservation of momentum requires that they travel if opposite directions. A simultaneous detection of gamma ray photons in two detectors places the source on a line between those detectors.
![]() |
For a given location, you can sum the signal from all detector pairs that correspond to a line going through that location.
All directions are equally probable for a given location, so you can normalize the signal as a measure of the concentration of the radioisotope at that location.
|
PET scans are increasing in use for all parts of the body, but have been of particular value for imaging of the brain. Getting a radioisotope into the brain for measurement is challenging because the protection of the blood-brain barrier makes it difficult to get most substances into the brain. A positron emitter that can be inserted into a glucose molecule is the fluorine isotope 18F. Not only does the glucose pass the blood-brain barrier and enter the brain easily, the concentration of the radioactively tagged glucose is a measure of the level of metabolic activity at that location in the brain.
The PET scan then becomes a tool for fundamental research in that it can provide a map of the level of metabolic activity in the brain. It has been used to map the different patterns of brain activity for various activities, for example listening to music versus doing a math problem. It can map the areas of hyperactivity associated with seizure activity. Patterns of activity associated with certain brain disorders may be identified.
Another major benefit of the PET scan is in the diagnosis and treatment of cancer. An area of abnormally high activity might be suspected to be a fast-growing malignancy. If the cancer is treated with radiation or chemotherapy, the PET scan is again valuable because it is the only practical way to determine whether the location of a tumor is now metablolically inactive as a result of the therapy, or is still consuming glucose as an indication of continued activity and failure of the therapy.
Nuclear Magnetic Moments
Associated with each nuclear spin is a magnetic moment which is associated with the angular momentum of the nucleus. It is common practice to express these magnetic moments in terms of the nuclear spin in a manner parallel to the treatment of the magnetic moments of electron spin and electron orbital angular momentum.
For the electron spin and orbital cases, the magnetic moments are expressed in terms of a unit called a Bohr magneton which arises naturally in the treatment of quantized angular momentum.
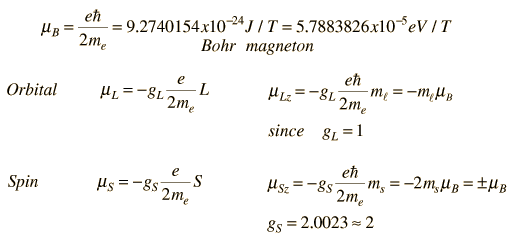
Generally, the measured quantity is proportional to the z-component of the magnetic moment (the component along the experimentally determined direction such as the direction of an applied magnetic field, etc. ). In this treatment, the use of a "gyromagnetic ratio" or "g-factor" is introduced. The g-factor for orbital is just gL = 1, but the electron spin g-factor is approximately gS = 2.
For the nuclear case we proceed in a parallel manner. The nuclear magnetic moment is expressed in terms of the nuclear spin in the form



Neutron: g = -3.8260837 +/- 0.0000018
The proton g-factor is far from the gS = 2 for the electron, and even the uncharged neutron has a sizable magnetic moment! For the neutron, this suggests that there is internal structure involving the movement of charged particles, even though the net charge of the neutron is zero. If g=2 were an expected value for the proton and g=0 were expected for the neutron, then it was noted by early researchers that the the proton g-factor is 3.6 units above its expected value and the neutron value is 3.8 units below its expected value.
This approximate symmetry was used in trial models of the magnetic moment, and in retrospect is taken as an indication of the internal structure of quarks in the standard model of the proton and neutron.Note that the maximum effective magnetic moment of a nucleus in nuclear magnetons will be the g-factor multiplied by the nuclear spin. For a proton with g = 5.5857 the quoted magnetic moment is m = 2.7928 nuclear magnetons.
Nuclide | spin I | m in mN |
-1.9130418 | ||
+2.7928456 | ||
+0.8574376 | ||
-1.89279 | ||
+0.09062293 | ||
+4.733 | ||
+6.1705 |
Nuclear Magnetic Resonance
When the nuclear magnetic moment associated with a nuclear spin is placed in an external magnetic field, the different spin states are given different magnetic potential energies. In the presence of the static magnetic field which produces a small amount of spin polarization, a radio frequency signal of the proper frequency can induce a transition between spin states.
This "spin flip" places some of the spins in their higher energy state. If the radio frequency signal is then switched off, the relaxation of the spins back to the lower state produces a measurable amount of RF signal at the resonant frequency associated with the spin flip. This process is called Nuclear Magnetic Resonance (NMR). A magnetic dipole moment (usually just called "magnetic moment") in a magnetic field will have a potential energy related to its orientation with respect to that field.
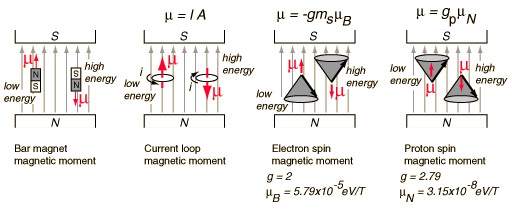
Note that the electron spin magnetic moment is opposite to the electron spin while the proton spin magnetic moment is in the direction of the proton spin. The electron spin or proton spin will tend to precess around the magnetic field with a frequency traditionally called the Larmor frequency. For a 1 Tesla magnetic field this Larmor frequency would be
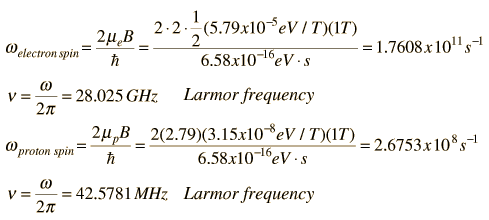
The Larmor frequency can be visualized classically in terms of the precession of the magnetic moment around the magnetic field, analogous to the precession of a spinning top around the gravity field. It can also be visualized quantum mechanically in terms of the quantum energy of transition between the two possible spin states for spin 1/2.
This can be expressed as a photon energy according to the Planck relationship. The magnetic potential energy difference is hn = 2mB. The short table of Larmor frequencies below is from Hobbie, Ch 17 and Becker. An extensive list including the magnetic moments and Larmor frequencies of most elements can be found in Appendix A of Becker.
Particle | Spin | s-1T-1 | |
Electron | |||
Proton | |||
Deuteron | |||
Neutron | |||
The Larmor frequency of the electron spin is in the microwave region of the electromagnetic spectrum and is used in electron spin resonance.
The precession of the proton spin in the magnetic field is the interaction which is used in proton NMR. As a practical technique, a sample containing protons (hydrogen nuclei) is placed in a strong magnetic field to produce partial polarization of the protons.
A strong RF field is also imposed on the sample to excite some of the nuclear spins into their higher energy state. When this strong RF signal is switched off, the spins tend to return to their lower state, producing a small amount of radiation at the Larmor frequency associated with that field. The emission of radiation is associated with the "spin relaxation" of the protons from their excited state. It induces a radio frequency signal in a detector coil which is amplified to display the NMR signal.
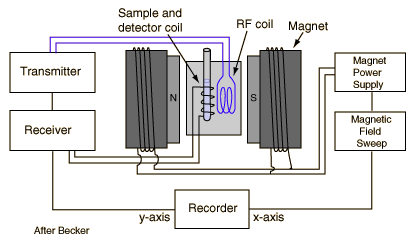
Since the Larmor frequency of the detected signal is proportional to the applied magnetic field, changing the magnitude of that field produces a different detected frequency. Placing a magnetic field gradient across a sample allows you to locate the source of the proton NMR signal in the sample. This is used to great advantage in the medical imaging process known as Magnetic Resonance Imaging.
Magnetic field
MRI scans require a magnetic field with two properties, uniform field density and strength. The magnetic field cannot vary more than 1/10,000 of 1% and field strength ranges (depending on the scanner) from 0.2 to 3 teslas in strength in scanners currently used clinically, with research scanners investigating higher field strengths such as 7 teslas.
The lower field strengths can be achieved with permanent magnets, which are often used in "open" MRI scanners for claustrophobic patients. Higher field strengths can be achieved only with superconducting magnets. An MRI with a 3.0 tesla strength magnet may be referred to as a "3-T MRI" or "3-tesla MRI"
Since the gradient coils are within the bore of the scanner, there are large forces between them and the main field coils, producing most of the hammering noise that is heard during operation. Without efforts to damp this noise, it can approach 130 decibels (dB) with strong fields (see also the subsection on acoustic noise).
Reflecting the fundamental importance and applicability of MRI in medicine, Paul Lauterbur of the University of Illinois at Urbana-Champaign and Sir Peter Mansfield of the University of Nottingham were awarded the 2003 Nobel Prize in Physiology or Medicine for their "discoveries concerning magnetic resonance imaging".
Proton therapy
Proton therapy has shown promise in treating several kinds of cancer. Research of proton therapy continues, as doctors try to determine who may benefit from proton therapy treatment. Proton therapy isn't widely available in the United States
Radiation therapy is a type of cancer treatment that uses beams of intense energy to kill cancer cells. Radiation therapy most often gets its power from X-rays, but the power can also come from protons or other types of energy.
The term "radiation therapy" most often refers to external beam radiation therapy. During this type of radiation, the high-energy beams come from a machine outside of your body that aims the beams at a precise point on your body. During a different type of radiation treatment called brachytherapy (brak-e-THER-uh-pee), radiation is placed inside your body.
Radiation therapy damages cells by destroying the genetic material that controls how cells grow and divide. While both healthy and cancerous cells are damaged by radiation therapy, the goal of radiation therapy is to destroy as few normal, healthy cells as possible.
History
The first suggestion that energetic protons could be an effective treatment method was made by Robert R. Wilson in a paper published in 1946 while he was involved in the design of the Harvard Cyclotron Laboratory (HCL).[7] The first treatments were performed with particle accelerators built for physics research, notably Berkeley Radiation Laboratory in 1954 and at Uppsala in Sweden in 1957. In 1961, a collaboration began between HCL and the Massachusetts General Hospital (MGH) to pursue proton therapy.
Over the next 41 years, this program refined and expanded these techniques while treating 9,116 patients before the Cyclotron was shut down in 2002.
The world's first hospital-based proton therapy center was a low energy cyclotron centre for ocular tumours at the Clatterbridge Centre for Oncology in the UK, followed in 1990 at the Loma Linda University Medical Center (LLUMC) in Loma Linda, California.
Later, The Northeast Proton Therapy Center at Massachusetts General Hospital was brought online, and the HCL treatment program was transferred to it during 2001 and 2002.
By 2010 these facilities were joined by an additional seven regional hospital-based proton therapy centers in the United States alone, and many more worldwide.
Comparison with other treatment options
The issue of when, whether, and how best to apply this technology is controversial. As of 2012 there have been no controlled trials to demonstrate that proton therapy yields improved survival, or other clinical outcomes (including impotence in prostate cancer) compared to other types of radiation therapy, although a 5-year study of prostate cancer is underway at Massachusetts General Hospital.
Proton therapy is far more expensive than conventional therapy. Using current technology, proton therapy requires a very large capital investment (from $100M to more than $180M). Preliminary results from a three-year 2009 study, including high dose treatments, show very few side effects.
Advanced Oncotherapy's device, known as LIGHT, costs around £25m compared with price tags exceeding £100m for existing machines. It is also more sophisticated than its rivals, according to Dr Mike Sinclair, chief executive of Advanced Oncotherapy. Photo:
The collaboration has slashed the cost of proton beam therapy, a type of cancer treatment that fires high-precision beams of particles at tumours, minimising damage to surrounding body tissue.
While the Large Hadron Collider is the world's biggest physics experiment, its technological advances have made it possible to shrink the size of equipment needed to accelerate protons to the speeds needed for blasting tumours.
AIM-listed Advanced Oncotherapy has been working with a spin-off from CERN, the group behind the Large Hadron Collider, to make the technology suitable for hospitals, and on Wednesday fully acquired the technology start-up.
The advance will allow thousands more cancer patients every year to benefit from proton beam therapy, which causes much less damage to healthy tissue than the standard radiation treatments using X-rays and other types of particle beams. Proton beam therapy is especially useful when tumours are in or near vital organs.
Six UK hospitals have already put in orders for the cancer-treating devices, and the first machines will be ready to use in 2016. Currently only one centre offers the cutting-edge treatment in Britain, as the cost of existing proton beam therapy machines is so high. Two US hospitals have also agreed to buy the machine.
Basics:
Nuclei with an odd mass or odd atomic number have "nuclear spin" (in a similar fashion to the spin of electrons). This includes 1H and 13C (but not 12C). The spins of nuclei are sufficiently different that NMR experiments can be sensitive for only one particular isotope of one particular element. The NMR behaviour of 1H and 13C nuclei has been exploited by organic chemist since they provide valuable information that can be used to deduce the structure of organic compounds. These will be the focus of our attention.
Since a nucleus is a charged particle in motion, it will develop a magnetic field. 1H and 13C have nuclear spins of 1/2 and so they behave in a similar fashion to a simple, tiny bar magnet. In the absence of a magnetic field, these are randomly oriented but when a field is applied they line up parallel to the applied field, either spin aligned or spin opposed. The more highly populated state is the lower energy spin aligned situation. Two schematic representations of these arrangements are shown below:





The first suggestion that energetic protons could be an effective treatment method was made by Robert R. Wilson in a paper published in 1946 while he was involved in the design of the Harvard Cyclotron Laboratory (HCL).[7] The first treatments were performed with particle accelerators built for physics research, notably Berkeley Radiation Laboratory in 1954 and at Uppsala in Sweden in 1957. In 1961, a collaboration began between HCL and the Massachusetts General Hospital (MGH) to pursue proton therapy.
![]() |
Proton X-Ray images |
The world's first hospital-based proton therapy center was a low energy cyclotron centre for ocular tumours at the Clatterbridge Centre for Oncology in the UK, followed in 1990 at the Loma Linda University Medical Center (LLUMC) in Loma Linda, California.
Later, The Northeast Proton Therapy Center at Massachusetts General Hospital was brought online, and the HCL treatment program was transferred to it during 2001 and 2002.
By 2010 these facilities were joined by an additional seven regional hospital-based proton therapy centers in the United States alone, and many more worldwide.
Comparison with other treatment options
The issue of when, whether, and how best to apply this technology is controversial. As of 2012 there have been no controlled trials to demonstrate that proton therapy yields improved survival, or other clinical outcomes (including impotence in prostate cancer) compared to other types of radiation therapy, although a 5-year study of prostate cancer is underway at Massachusetts General Hospital.
Proton therapy is far more expensive than conventional therapy. Using current technology, proton therapy requires a very large capital investment (from $100M to more than $180M). Preliminary results from a three-year 2009 study, including high dose treatments, show very few side effects.
Advanced Oncotherapy's device, known as LIGHT, costs around £25m compared with price tags exceeding £100m for existing machines. It is also more sophisticated than its rivals, according to Dr Mike Sinclair, chief executive of Advanced Oncotherapy. Photo:
The collaboration has slashed the cost of proton beam therapy, a type of cancer treatment that fires high-precision beams of particles at tumours, minimising damage to surrounding body tissue.
While the Large Hadron Collider is the world's biggest physics experiment, its technological advances have made it possible to shrink the size of equipment needed to accelerate protons to the speeds needed for blasting tumours.
AIM-listed Advanced Oncotherapy has been working with a spin-off from CERN, the group behind the Large Hadron Collider, to make the technology suitable for hospitals, and on Wednesday fully acquired the technology start-up.
The advance will allow thousands more cancer patients every year to benefit from proton beam therapy, which causes much less damage to healthy tissue than the standard radiation treatments using X-rays and other types of particle beams. Proton beam therapy is especially useful when tumours are in or near vital organs.
Six UK hospitals have already put in orders for the cancer-treating devices, and the first machines will be ready to use in 2016. Currently only one centre offers the cutting-edge treatment in Britain, as the cost of existing proton beam therapy machines is so high. Two US hospitals have also agreed to buy the machine.
Nuclear Magnetic Resonance
Basics:
Nuclei with an odd mass or odd atomic number have "nuclear spin" (in a similar fashion to the spin of electrons). This includes 1H and 13C (but not 12C). The spins of nuclei are sufficiently different that NMR experiments can be sensitive for only one particular isotope of one particular element. The NMR behaviour of 1H and 13C nuclei has been exploited by organic chemist since they provide valuable information that can be used to deduce the structure of organic compounds. These will be the focus of our attention.
Since a nucleus is a charged particle in motion, it will develop a magnetic field. 1H and 13C have nuclear spins of 1/2 and so they behave in a similar fashion to a simple, tiny bar magnet. In the absence of a magnetic field, these are randomly oriented but when a field is applied they line up parallel to the applied field, either spin aligned or spin opposed. The more highly populated state is the lower energy spin aligned situation. Two schematic representations of these arrangements are shown below:




I |
n NMR, EM radiation is used to "flip" the alignment of nuclear spins from the low energy spin aligned state to the higher energy spin opposed state. The energy required for this transition depends on the strength of the applied magnetic field (see below) but it is small and corresponds to the radio frequency range of the EM spectrum.

As this diagram shows, the energy required for the spin-flip depends on the magnetic field strength at the nucleus. With no applied field, there is no energy difference between the spin states, but as the field increases so does the separation of energies of the spin states and therefore so does the frequency required to cause the spin-flip, referred to as resonance.
|

The basic arrangement of an NMR spectrometer is shown to the left. The sample is positioned in the magnetic field and excited via pulsations in the radio frequency input circuit. The realigned magnetic fields induce a radio signal in the output circuit which is used to generate the output signal. Fourier analysis of the complex output produces the actual spectrum.The pulse is repeated as many times as necessary to allow the signals to be identified from the background noise.
|
Sem comentários:
Enviar um comentário